Introduction
According to the Food and Agriculture Organization, the number of people facing food scarcity increased by over 150 million in 2020 compared with that in the preceding year (Brenton et al., 2022). Traditional meat resources have raised concerns regarding sustainability owing to environmental issues and their low bioconversion rate for energy, highlighting the need for alternative protein sources (Park, 2021). Edible insects may constitute a valid alternative to vertebrates, and humans consume over 2,000 species of insects worldwide (van Huis, 2016). Edible insects are rich in high-quality protein, vitamins, and amino acids; moreover, they emit less greenhouse gas per unit of protein than livestock and are more cost-effective (Aguilar-Toalá et al., 2022; Tang et al., 2019). The mealworm (Tenebrio molitor), an insect belonging to the Tenebrionidae (Coleoptera) family, is one of the most well-recognized edible insects globally (Peng et al., 2019). It has been approved as a food item in numerous countries, including South Korea, China, Japan, the United States, the Netherlands, and Belgium (Yoo et al., 2011). Furthermore, mealworms are rich in proteins and unsaturated fatty acids (Gkinali et al., 2022; Ravzanaadii et al., 2012).
To utilize edible insects as a food item, establishing appropriate sacrifice methods is crucial. The sacrifice process, that is, the killing of live insects through pretreatment, is the initial step to preparing insect-based food (Gnana Moorthy Eswaran et al., 2023). This process also focuses on the inactivation of microorganisms and innate enzymes (Grabowski and Klein, 2017); without proper sacrifice procedures, decomposition is accelerated during storage. Various sacrifice methods can be applied to edible insects. For example, Larouche et al. (2019) investigated blanching, mechanical disruption, heating, freezing, and asphyxiating in black soldier fly (Hermetia illucens) larvae. However, a standardized industrial method for sacrificing insects has yet to be established, and relevant previous studies are lacking. In addition, the quality of the end products generated by sacrificed mealworms has been disregarded.
Insect appearance can evoke aversion among consumers; thus, powdering is frequently employed to address this issue (Sogari et al., 2023). In general, insect pulverization is conducted after dehydration; it reduces the insects’ water activity, thereby hindering spoilage (Krokida and Marinos-Kouris, 2003; Son et al., 2019). Additionally, powdered insects can be applied to diverse food products, such as muffins and bread (Khuenpet et al., 2020). On the other hand, the overall quality of sacrificed insects after cooking is also an important feature. When whole sacrificed insects are distributed, consumers need to cook them before eating. Therefore, considering the characteristics of pulverized or cooked products is imperative when evaluating sacrifice methods.
This study aimed to establish a suitable sacrifice method for live mealworm larvae based on five sacrifice techniques: freezing, sonication, blanching, post-blanching mid-infrared irradiation, and roasting. After sacrifice, mealworm larvae were processed into powder form and their compositional and physicochemical properties evaluated. Additionally, we examined the post-cooking characteristics of the sacrificed mealworms to evaluate their suitability as food items. Ultimately, we endeavored to establish an optimal sacrifice method for mealworms and examine the characteristics of their end products. We anticipate that this study’s findings will contribute to the development of a standardized protocol for sacrificing insects, ultimately enhancing their quality for human consumption.
Materials and Methods
All chemicals used in this study were of analytical grade and purchased from Sigma-Aldrich (St. Louis, MO, USA). The mealworm larvae used in this study were procured from a farm near Gyeonggi-do, where mealworms are reared for human consumption.
The mealworm larvae were fasted for 3 days, washed 2–3 times with clean water, and prepared by removing excess moisture with paper towels. Freezing sacrifice (F) was performed at –20°C for 3 days, while sonication sacrifice (S) was conducted at 50°C for 6 min (JAC-5020, Kodo, Hwaseong, Korea). Blanching sacrifice (B) was conducted by immersing the larvae in boiling water (95°C–100°C) for 6 min. Boiling water—at a weight tenfold that of the mealworms—was prepared for blanching. In the other sample group, the blanched mealworms were further treated with mid-infrared irradiation (BI). An MS3-6 industrial mid-infrared irradiation device (Lichtzen, Gunpo, Korea) was used, and the samples were irradiated at 110°C for 6 min. Roasting sacrifice (R) was conducted at 150°C for 6 min using a pan. The differentially sacrificed mealworms (five groups: F, S, B, BI, and R) were subsequently dried at 60°C for 12 h using an industrial hot air dryer (LH.FC-PO-150, Lab House, Pocheon, Korea) and ground into powder using an industrial pin mill (GRC-10, Garyeo, Siheung, Korea).
Sample proximate composition was measured using the methods prescribed by the AOAC (2000; methods 932.06, 925.09, and 923.03). Carbohydrate content was calculated by subtracting the moisture, crude protein, crude fat, and ash contents from the entire proportion.
The free amino acid content of the samples was measured using the method of Godel et al. (1984), with modifications. Mealworm powder was mixed with distilled water for extraction (1:10, w/v) and sonicated at 40°C for 30 min. After centrifugation at 2,000×g for 20 min (Combi 514R, Hanil Science Industrial, Incheon, Korea), the supernatant was collected and extraction process repeated twice. The combined supernatant was filtered through a 0.2 μm polytetrafluoroethylene filter. The prepared analysis samples and amino acid standards were each mixed with borate buffer, fluorenylmethyloxycarbonyl chloride, and o-phthaldialdehyde/2-mercaptopropionic acid and subsequently injected into a high-performance liquid chromatography system for analysis. The analysis conditions are shown in Table 1.
Sample color was measured using a colorimeter (CM-3500d, Minolta, Tokyo, Japan) by placing 4 g of powdered sample in a 35Φ petri dish (SPL Life Sciences, Pocheon, Korea). The light source conditions were set to D65-10°, and each sample was measured five times, with the results expressed using the CIE L*, CIE a*, and CIE b* color system, wherein CIE L*, CIE a*, and CIE b* signify lightness, redness, and yellowness, respectively.
Water-adhesion capacity (WAC) and oil-adhesion capacity (OAC) measurements were conducted following the method of Cho et al. (2013). Briefly, 10 mL of distilled water or soybean oil (Sajo, Seoul, Korea) was placed in a 15 mL tube, and 0.5 g of mealworm powder was added. The mixture was maintained at 20°C for 1 h, with vigorous mixing every 15 min. After an hour, the mixture was centrifuged at 1,600×g for 25 min using a centrifuge (Combi-514R, Hanil Science Industrial, Gimpo, Korea). The supernatant was subsequently removed and the weight of the remaining residue measured. WAC and OAC were calculated by comparing the weight of the dried powder with that of the final residue and expressed as the amount of absorbed water or oil per gram of powder.
Sample acceptance was evaluated by 30 untrained students from Seoul National University. Random numbers were assigned to each sample, and the samples were presented in a randomized order. Participants were instructed to rinse their mouths with water after tasting each sample. Acceptance was evaluated using a 7-point scale, where 1 indicated “extremely dislike,” 4 indicated “neither like nor dislike,” and 7 indicated “extremely like,” with higher scores reflecting greater acceptance. The sensory acceptance of the powder was assessed based on four attributes: appearance, flavor, texture, and overall acceptance. The consumer acceptance study was approved by the Institutional Review Board (IRB) of Seoul National University (IRB no. 1610/001-005).
The blanching-sacrificed mealworm larvae were prepared using the procedure described in Sacrifice Conditions. After sacrifice, they were placed on a sieve for 30 min to remove excess water and subsequently dabbed with paper towels to eliminate surface moisture. They were cooked using four different methods: steaming (C-S), boiling (C-B), panfrying (C-PF), and deep-fat frying (C-DF). For C-S, a steamer (WMF Steamer, WMF, Geislingen, Germany) was used to cook the larvae at 90°C–95°C for 10 min. C-B samples were prepared by cooking mealworms in boiling water for 3 min. C-PF samples were prepared by placing sacrificed mealworms in a pan and maintaining a consistent temperature of 200°C for 8 min. For C-DF, mealworms were cooked in soybean oil (Sajo) at 180°C for 1.5 min. The cooking time required to achieve an internal temperature >80°C in mealworms was determined based on a preliminary study (Ab Aziz et al., 2020). The prepared samples were immediately cooled to room temperature on paper towels and subsequently subjected to analysis and sensory evaluation.
Cooked mealworm color was measured by placing 30 whole larvae into a 35Φ petri dish (SPL Life Sciences), according to the method described above.
The mechanical texture of the cooked mealworms was measured using a cutting test, referring to studies by Barat et al. (2002) and Lee et al. (2015). The analysis was conducted using a texture analyzer (TA/XT2, Stable Micro Systems, Surrey, UK) equipped with an HDP/BSK probe in compression mode with the “return to start” setting. One cooked mealworm was placed onto the probe, and the test was repeated with 10 respective samples per sample group. The pre-test and test speeds were set at 1 mm/s, while the post-test speed was set at 5 mm/s. The trigger force was set at 20 g. The experiment was conducted at the BT Research Facility Center, Chung-Ang University. The peak force (N), cutting distance (mm), and total positive area (N×second) were measured.
To conduct quantitative descriptive analysis (QDA), 12 panelists with no aversion to mealworm and prior experience in QDA were recruited. After training and pre-evaluation, eight panelists were selected for the experiment. The panelists were recruited from Chung-Ang University (four men and four women). During training, the panelists familiarized themselves with the samples, and a 14-term lexicon was established through discussion. The lexicon encompassed the following categories: appearance (degree of darkness and gloss), odor (roasting odor, oily odor, mushroom odor, and steamed-grain aroma), flavor (roasted flavor, bitter taste, and savory taste), and texture (crispiness, chewiness, greasy, juiciness, and mouth coating). The selected terms were compared with those in the lexicon established for cooked mealworm larvae by Baek et al. (2015), and reference foods were utilized to train each sensory trait. In the QDA, each sample was assigned a random number and provided in random order. A 15 cm line scale was used to evaluate each sensory attribute. The QDA was approved by the IRB of Chung-Ang University (IRB no. 1041078-20230130-HR-021).
The volatile compound content of the cooked mealworm samples was analyzed using a gas chromatography-mass spectrometer (GC-MS), according to the method of Cheok et al. (2017) and Hiraide et al. (2004), with slight modifications. Mealworm samples (10 g) were mixed with 100 mL of distilled water and homogenized. After centrifugation at 3,000×g for 10 min, 5 mL of supernatant was transferred into a 20 mL headspace glass vial, and 3 g of sodium chloride was subsequently added. The vial was capped after purging with helium and heated at 80°C for 30 min in a headspace sampler, with shaking. The headspace sample was transferred to a GC (PerkinElmer 680 GC, PerkinElmer, Waltham, MA, USA) equipped with a VF-624ms column (60 m×0.530 mm i.d.×3.0 μm; Agilent Technologies, Santa Clara, CA, USA). The temperatures of the loop and transfer line were 180°C and 200°C, respectively, and the injector temperature was 250°C. The GC oven temperature, initially 35°C, was heated to 150°C at a rate of 5°C/min, reheated to 220°C at 10°C/min, and held for 5 min. The carrier gas was helium at a pressure of 200 kPa, and the inlet pressure was set to 100 kPa. The injection volume was 1.0 μL, and split mode was applied (2:1 split ratio). The separated volatile compounds were identified and quantified using MS (600T MS, PerkinElmer) at an ionization voltage of 70 eV. The volatile compounds in the mealworm samples were identified by comparing their mass spectra and retention times with authentic standards after matching with the National Institute of Standards and Technology (NIST08) database.
Statistical analyses were conducted using IBM SPSS Statistics (version 28, IBM, Armonk, NY, USA). To compare the sample groups, one-way analysis of variance was employed, followed by Duncan’s multiple-range test to identify statistical differences at a significance level of p<0.05. Experimental data are expressed as the mean±SD. Heatmap analysis was visualized using Prism software (version 9, GraphPad, La Jolla, CA, USA).
Results and Discussion
The proximate compositions of mealworm powders prepared using different sacrifice methods are presented in Table 2. The proximate composition of B was similar to that obtained in previous studies (Oliveira et al., 2024; Roncolini et al., 2019). F yielded the highest moisture content (10.49±0.69%, p<0.05), possibly because of the direct drying of the frozen sample with ice crystals on the surface. Moreover, the low temperature of the frozen sample potentially reduced the internal temperature of the dryer, lowering drying efficiency. S also exhibited a relatively high moisture content (3.48±0.11%), significantly higher than that of the heat-sacrificed samples, namely, B, BI, and R (p<0.05). The increased drying efficiency observed in the heat-treated samples potentially relates to structural changes in components such as the myofibrillar proteins of edible insects, which undergo heat-induced decomposition and deformation, thus reducing the water-retention capacity and enhancing drying efficiency (Shi et al., 2021). At the end of the experiment, BI and R displayed a higher moisture content than B (p<0.05), and this may be related to the surface hardening of BI and R owing to higher-temperature treatment (Koç et al., 2008). The crude fat content of BI and R was lower than that of B and S, indicating that dry-heat processes result in fat loss (p<0.05). Previous studies have also observed fat loss during the heating of edible insects (Muthee et al., 2024; Nyangena et al., 2020), and it may be caused by the expulsion of fat during heating. Conversely, B and BI yielded a lower crude protein content, suggesting that blanching reduces the product’s protein content (p<0.05). This phenomenon is commonly observed in protein-based foods because proteins leach into boiling water during blanching (Li et al., 2013). R exhibited the highest ash content (p<0.05), and BI, which had undergone both moist-heat and dry-heat processes, yielded the highest carbohydrate content (p<0.05). The increased carbohydrate content of B, BI, and R compared with that of F and S was considered to result from lipid and protein loss during heat treatment. Mealworms contain low amounts of simple sugars (approximately 3% of total soluble sugars), and chitin derivatives occupy a vast portion (Son et al., 2021). Owing to their considerable heat stability and low solubility in water and lipids, chitin derivatives may effectively have minimized carbohydrate loss of mealworms.
Free amino acid content is a key indicator directly influencing the taste of protein-based foods, as different free amino acid types contribute to various flavors, such as sweetness, bitterness, umami, saltiness, and sourness (Kong et al., 2017). For example, alanine, glycine, serine, and threonine impart a sweet taste, whereas arginine, histidine, isoleucine, leucine, lysine, methionine, phenylalanine, proline, and tyrosine potentially confer a bitter taste. Aspartic and glutamic acids are representative amino acids that possess an umami taste. Although we cannot determine the taste of foods based on free amino acid content alone owing to its complexity and the effect of other savoring compounds, the free amino acid content and composition substantively affect the taste of foods (Sirisena et al., 2024). Additionally, the free amino acid content provides valuable insights into protein leaching, degradation, and other modifications during processing. F had the highest free amino acid content (9,734.47±41.08 mg/100 g dry weight) among the five samples (p<0.05; Table 3). This accounted for approximately 9.7% of the total sample weight. Considering that the crude protein content of F was 49.05%, nearly 20% of the total protein content was determined to comprise free amino acids from F. In comparison, the proportion of free amino acids in the total protein is typically low in larger meats, such as raw pork, beef, and chicken, ranging from approximately 0.5% to 1.0% (Franco et al., 2010; Han et al., 2003). Additionally, in boiled soybeans, a major plant-based protein source, the free amino acid proportion is approximately 1.0% (Dajanta et al., 2011). Therefore, compared with other protein sources, freeze-sacrificed mealworms exhibited a remarkably high proportion of free amino acids. This suggests that using mealworms as a food ingredient, even in minute amounts, could significantly enhance the flavor of dishes owing to their rich free amino acid content. However, further research is required to evaluate the effect of mealworms on food taste, specifically in relation to their free amino acid content and other key taste components.
When mealworms were sacrificed using sonication, less than 5% of the free amino acids were lost; however, blanching and roasting resulted in approximately 90% and 80% free amino acid loss, respectively. This loss likely emanated from the leaching of free amino acids during the sacrifice process or their conversion into other compounds. After blanching, further treatment with medium-wave infrared irradiation increased the free amino acid content more than double. This suggests that incorporating medium-wave infrared treatment potentially enhances flavor acceptance owing to the increased free amino acid content.
Higher powder WAC and OAC values indicate better compatibility with solvents, rendering them more suitable for food applications owing to improved processability (Barbut, 1996). The WAC and OAC values of the powders are shown in Table 4. F and S exhibited significantly lower WAC values than the other samples (p<0.05), suggesting that their hydration capacity decreased with water addition. Sample B, prepared via moist-heat sacrifice, yielded the highest WAC value (p<0.05). This indicates that heat-treated samples have higher water affinity than non-heat- or less-heat-treated samples, with moist-heat sacrifice further enhancing water affinity. In terms of OAC, the S displayed noticeably lower values than the other samples, while the difference between the moist- and dry-heat sacrifice methods was not statistically significant (p<0.05). Previous studies have yielded mealworm powder WAC and OAC values of 0.80–1.79 and 0.60–1.58 g/g, respectively, exhibiting ranges similar to those obtained in our study (Borremans et al., 2020; Bußler et al., 2016; Stone et al., 2019).
The color values of the samples are presented in Table 4. Significantly low CIE L*, CIE a*, and CIE b* values were observed in the non-heat- and less-heat-sacrificed samples (F and S) compared with those in the heat-sacrificed samples (B, BI, and R; p<0.05). This indicates that the F and S powders exhibited darker and more achromatic colors. According to Leni et al. (2019), because mealworms possess a substantial amount of browning enzymes, such as tyrosinase, heat treatment can impede browning reactions in mealworms by inactivating enzymes. Among the heat-sacrificed samples, B and R generated similar CIE L*, CIE a*, and CIE b* values, suggesting that the moist- and dry-heat sacrifice methods did not cause significant color differences.
Sample acceptance was evaluated using a 7-point scale divided into the following categories: appearance, flavor, texture, and overall acceptance (Table 5). F and S yielded lower acceptance scores across all categories than the heat-treated samples (B, BI, and R), with F generating the lowest (p<0.05). Among the heat-treated samples, B and R produced similar acceptance scores across all categories. BI demonstrated noticeably higher acceptance scores for appearance, flavor, and overall acceptance than the other samples. The appearance and flavor differences are presumably associated with BI’s higher CIE L* value and higher free amino acid content, respectively.
On verifying the quality of mealworm powders processed using different sacrifice methods, blanching yielded the most suitable characteristics for industrial use. Therefore, we further examined the blanching-sacrificed mealworms, evaluating their post-cooking characteristics.
The samples cooked using moist-heat methods (C-S and C-B) generated cooking loss values of –2.21±0.83% and –10.75±0.77%, respectively, signifying increased weight after cooking (Table 6). In contrast, C-PF and C-DF yielded cooking loss values of 58.02±1.07% and 58.36±0.67%, respectively. Yoo et al. (2002) reported that alterations in food weight are heavily influenced by cooking method, size, temperature, and time. Therefore, although the sample size remained constant in this study, cooking method, temperature, and duration differences probably accounted for the cooking loss. McWilliams (2001) suggested that the lack of cooking loss associated with moist-heat cooking emanates from minimal dehydration during the process. However, in several foods, even moist-heat cooking can result in substantial cooking losses because of fiber contraction and muscle denaturation during cooking, thereby reducing water retention capacity (Latorre et al., 2019). The post-cooking weight gain of C-B and C-S suggests that the minimal leaching of internal components during cooking may result from their chitinous exoskeleton, which remains structurally stable under heat and prevents significant loss of internal components (Jang et al., 2004). Additionally, mealworms contain minute quantities of low-molecular-weight sugars, which easily leach from other foods (Son et al., 2021). This explains why C-B, which allowed more water penetration during cooking, exhibited greater post-cooking weight gain than C-S (p<0.05). The dry heat-cooked samples also displayed minimal internal component leaching. Although they exhibited approximately 58% cooking loss, the pre-cooking moisture content of the mealworms (55%–60%) suggests that mostly water was lost, and marginal leaching of other components occurred.
In terms of color values, moist heat-cooked mealworms (C-S and C-B) displayed a brighter color than dry heat-cooked mealworms (C-PF and C-DF; p<0.05; Table 6), and this may be related to an attenuated Maillard reaction owing to a lower cooking temperature (Grossmann et al., 2021). Regarding CIE a* and CIE b*, the dry heat-cooked mealworms yielded higher CIE a* and lower CIE b* values (p<0.05). Chin et al. (2012) reported that a lower sample moisture content leads to an increase in the CIE a* value, and an enhanced Maillard reaction may also elevate the CIE a* of C-PF and C-DF.
To assess the mechanical texture of the cooked mealworms, hardness, brittleness, and the total force until cutting were evaluated using a cutting test (Table 6). C-DF yielded the highest hardness value (7.82±1.65 N/mm2; p<0.05), while no significant differences were observed among the other three samples. In addition to hardness, the brittleness of the mealworm samples was determined because crispness is a critical textural characteristic of mealworms. Brittleness effectively reflects the viscoelastic properties of a sample; a lower brittleness value indicates higher viscosity, whereas a high brittleness value is related to a crispy texture (Zoulias et al., 2002). On comparing the brittleness values of the four samples, C-S and C-B produced similar values (15.58±1.44 and 14.25±2.61 N/mm, respectively), with no significant difference (p>0.05). In contrast, the C-PF sample yielded a significantly higher value (34.48±6.22 N/mm) than the C-S and C-B samples (p<0.05), while the C-DF sample generated the highest value (58.19±12.28 N/mm; p<0.05). These results suggest that dry heat-cooked mealworms have a crispier texture than moist heat-cooked mealworms. The total force until cutting reflects chewy and mouth-coating traits. It was considerably greater in moist heat-cooked samples than in dry heat-cooked samples (p<0.05), and sensory evaluation was anticipated to confirm this.
QDA of the cooked mealworm samples was conducted using a 14-term lexicon (two terms for appearance, four for odor, three for flavor, and five for texture), and its results are presented in Table 7. Significant differences were observed between moist and dry heat-cooked samples across all attributes (p<0.05). In the moist heat-cooked samples (C-S and C-B), no significant differences were found in sensory attributes, except for the steamed-grain odor. This indicates that the steaming and boiling methods result in minimal differences in the sensory characteristics of cooked mealworms. Steaming, however, enhanced steamed-grain odor intensity, possibly because of the leaching of water-soluble aromatic compounds into the cooking water during boiling. In contrast to the minimal sensory differences between C-B and C-S, dry heat-cooked mealworms (C-PF and C-DF) displayed significant differences across most sensory attributes, except for crispiness, juiciness, and mouth-coating. Dry heat-cooked samples appeared darker, a phenomenon attributed to water loss and enhanced browning reactions at higher cooking temperatures (Grossmann et al., 2021). C-DF yielded the highest gloss value (12.63±0.64) as well as the highest values in oil-related attributes, such as oily odor and greasiness (p<0.05). Regarding odor properties, steamed-grain and mushroom odors were significantly more pronounced in moist heat-cooked mealworms (p<0.05), indicating that different cooking methods cause distinct flavor profiles. In dry heat-cooked mealworms, these odors attenuated, whereas roasting odor, nutty flavor, and roasted flavor intensified. In terms of texture, moist heat-cooked mealworms exhibited increased chewiness, juiciness, and mouth-coating properties. In contrast, dry-heat cooking enhanced crispy and greasy textures. The sensory differences observed between moist- and dry-heat cooking are consistent with those reported by Baek et al. (2015), who also highlighted distinct sensory attributes based on the cooking method. The intensified steamed-grain and mushroom odors in moist heat-cooked mealworms suggests the potential application of mealworms as an ingredient of broths requiring such flavor profiles.
Sample volatile compound content was analyzed using GC–MS to examine the odorous characteristics of cooked mealworms (Fig. 1). A total of 12 volatile compounds were identified in the samples, and C-PF yielded the highest peak areas across all volatile compounds. Cooking temperature substantially affected the types and amounts of volatile compounds in mealworms, an aspect potentially related to the Maillard reaction rate (Żołnierczyk and Szumny, 2021). Likewise, the C-DF sample also contained larger amounts of volatile compounds than C-S and C-B. Among the volatile compounds, 2-methylbutanal and isobutyraldehyde generated the highest peak areas in the C-PF sample. Reportedly, 2-methylbutanal possesses chocolate, musty, and nutty aromas, while isobutyraldehyde has a caramel-like aroma (Cai et al., 2023; Perez-Santaescolastica et al., 2022). Moreover, Sohail et al. (2022) stated that 2,5-dimethyl-pyrazine and trimethyl-pyrazine can confer roasted aromas to foods. Therefore, these volatile compounds potentially lend distinctive odor characteristics to C-PF samples. This study’s volatile compound analysis in cooked mealworms was limited by the compounds’ undetectably weak odor. Therefore, only 12 volatile compounds were identified in the present study. A more effective headspace preparation method is required to comprehensively detect volatile compounds in mealworm samples.
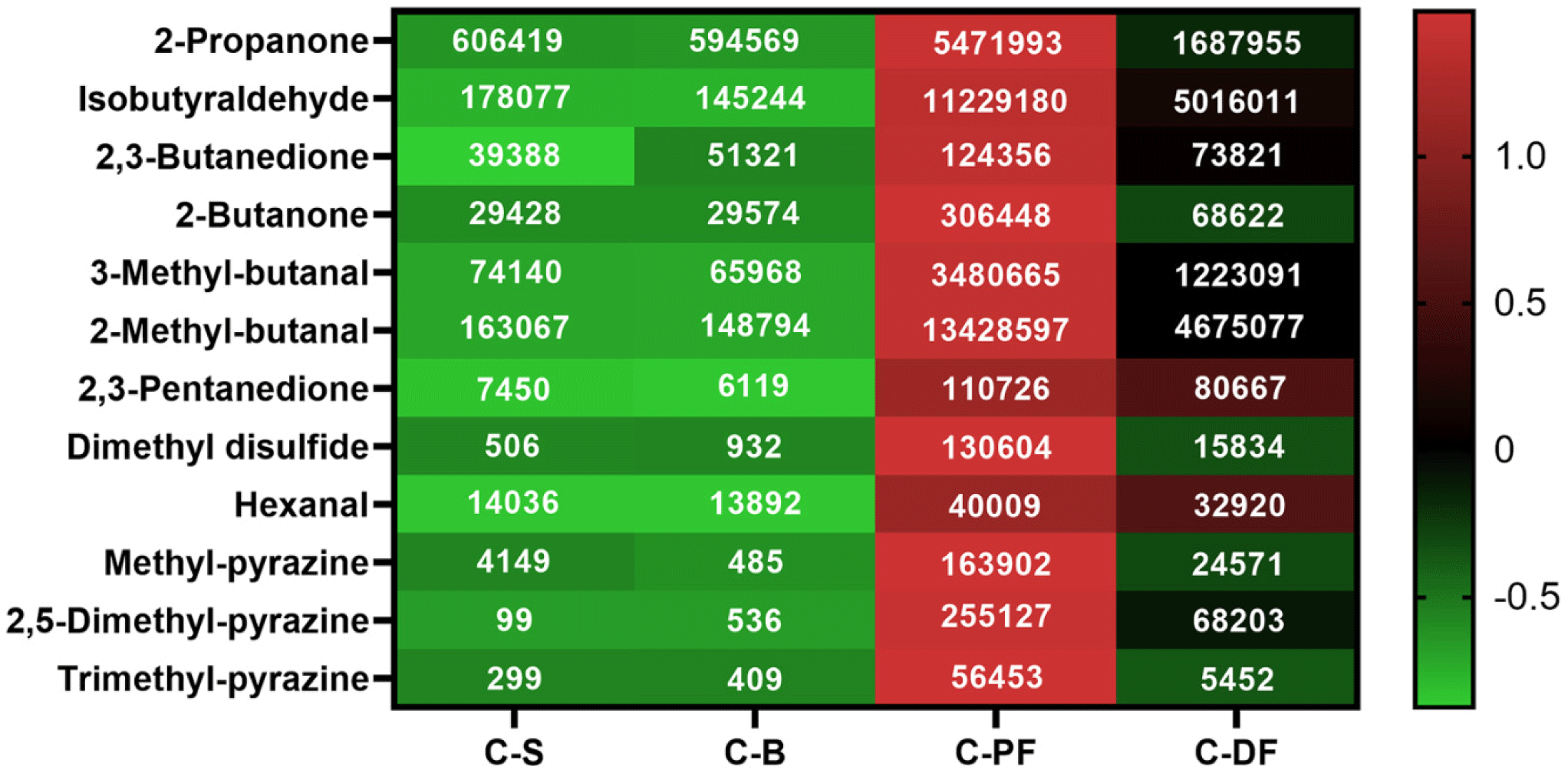
Conclusion
This study purposed to establish an appropriate mealworm sacrifice method and evaluate its post-cooking characteristics. When mealworms were sacrificed using non-heat and less-heat methods (freezing and sonication), they retained free amino acids efficiently; however, these processes reduced the drying efficiency, WAC, and acceptance scores. In contrast, heat-based sacrifice methods (blanching and roasting) increased the physicochemical properties and consumer acceptance of mealworm powders. Compared with roasting, blanching yielded higher WAC and OAC values, signifying superior usability as a food ingredient. In addition, we found post-blanching mid-infrared irradiation treatment to improve consumer acceptance. Meanwhile, we also analyzed the post-cooking characteristics of blanching-sacrificed mealworms in order to increase their applicability as a food item. Moist-heat cooking methods (steaming and boiling) increased the CIE L* and CIE b* of mealworms and presented a strong chewy, juicy, and mouth-coating texture with steamed-grain and mushroom odors. Conversely, dry heat-cooked (panfrying and deep-fat frying) mealworms yielded a high CIE a* value and crispy texture. According to the sensory analysis, dry heat-cooked mealworms produced high roasting odor and savory taste scores. Moreover, C-PF yielded a distinctive, intense aroma based on the prolific volatile compound content. In summary, we verified the strengths of blanching as a mealworm sacrifice method by comparing it with other sacrifice methods and also revealed its post-cooking characteristics. However, we could not devise novel technology for sacrificing and cooking mealworms, and blanching carries certain limitations when dealing with bulk quantities. Therefore, to identify the optimal mealworm sacrifice method, further research is warranted.