Introduction
Nanoparticles are nano-sized colloidal delivery systems that can be used for the encapsulation, protection, and delivery of bioactive compounds (Chen et al., 2006; Muthu and Wilson, 2012). Due to their subcellular size (< 200 nm), nanoparticles have more surface areas interacting with the mucosa of small intestine compared with microparticles (> 1,000 nm). It can extend the gastrointestinal transit time and improve the bioavailability of encapsulated bioactive compounds (Chen and Subirade, 2005; Chen et al., 2006; Duchêne and Ponchel, 1997). It has been suggested that the physicochemical properties of nanoparticles, especially particle size, can be important factors affecting the bioavailability of encapsulated bioactive compounds since smaller size with larger surface areas may increase the transit time in the digestive tract, bonding to and reaction with biological cells, as well as cellular uptake of nanoparticles (Ha et al., 2013; Ha et al., 2015; Win and Feng, 2005; Zhang et al., 2008). To enhance the cellular uptake and bioavailability of encapsulated bioactive compounds, it is important to develop new manufacturing process, which can modulate the physicochemical properties of beta-lactoglobulin (β-lg) nanoparticles.
Recently, there have been great interests in the development of delivery systems for food application using food-based materials instead of synthetic polymers (Chen et al., 2006; Patel and Velikov, 2011). Among food-based materials, food proteins including β-lg have been used to produce delivery systems due to their GRAS (generally recognized as safe) status, high nutritional value, and various functional properties. β-lg is a globular whey protein with a molecular weight of 18,400 Da and composed of 162 amino acids. Since it is biodegradable and has abilities to bind various hydrophobic bioactive compounds and to form gels, β-lg has been regarded as a potential material for food-based delivery systems (Chen and Subirade, 2005; Zimet and Livney, 2009). Various manufacturing processes, such as spray-drying (Bruschi et al., 2003), coacervation (Mauguet et al., 2002), and emulsifying-cross-linking (Lee et al., 2013), have been developed to produce food protein-based delivery systems. However, these processes require the high heat-treatment (e.g., spray-drying, > 100°C) or the use of organic solvents and toxic cross-linking agent (e.g., glutaraldehyde), which can lead to the destruction of heat-sensitive bioactive compounds encapsulated in delivery system, as well as toxicity problems induced with residual organic solvents, respectively (Birnbaum et al., 2000; Chen et al., 2006). For the production of delivery system to minimize those potential problems, it is necessary to develop food protein-based delivery systems under mild heating condition (i.e., <75°C) with GRAS delivery materials.
It has been suggested that heat treatment is the one of simple processes to form whey protein-based delivery systems (Leclerc et al., 2005; Lee et al., 2013). Heat treatment (e.g., above 60°C), which affects the secondary, tertiary, and quaternary structure of whey proteins (Hoffmann and van Mil, 1997; Relkin, 1998), has been widely used to induce the denaturation and aggregation of whey proteins (Hongsprabhas and Barbut, 1996). On the other hand, sub-ambient temperature treatment below 20°C induces the conformational changes of β-lg (Davidovic et al., 2009; Prabakaran and Damodaran, 1997). It can lead to intermolecular associations between whey protein molecules via hydrophobic interactions and disulfide linkages resulting in the formation of whey protein-based delivery systems (Ha et al., 2015; Hoffmann and van Mil, 1997; Lee et al., 2013). The heat-induced gelation and aggregation of β-lg may consist of complex multiple steps (i.e., initiation, propagation, and termination) (Prabakaran and Damodaran, 1997). The conformational changes of β-lg may be involved in an initial step for heat-induced denaturation and aggregation of β-lg (Hoffmann and van Mil, 1997; Prabakaran and Damodaran, 1997). Since the conformational changes of β-lg can be promoted at alkaline pH (e.g., pH 9.5) (Monahan et al., 1995), the use of sub-ambient temperature and mild heat temperature treatment at pH 9.5 may contribute to more extensive conformational changes of β-lg affecting chemical interactions in protein networks. Structural changes in the exposure of buried free thiol and hydrophobic groups of β-lg resulting from heat treatment may lead to the polymerization of β-lg molecules due to the formation of disulfide bonds and hydrophobic associations (Ha et al., 2013; Prabakaran and Damodaran, 1997), which can affect the physicochemical properties of β-lg nanoparticles. Therefore, understanding the conformational changes of β-lg at pH 9.5 during thermal-induced unfolding and aggregation is critical for developing simple but effective manufacturing processes to modulate the physicochemical properties of β-lg nanoparticles, especially particle size.
The objectives of this research are to manufacture β-lg nanoparticles using two-step temperature process and to determine the impacts of two-step temperature treatments on the structural changes and physicochemical properties of β-lg nanoparticles.
Materials and Methods
β-lg was generously supplied by Davisco International, Inc. (Le Sueur, USA). Calcium chloride (CaCl2), 1-anilinonaphthalene-8-sulfonic acid (ANS), ethylene diamine tetra acetic acid (EDTA), 5,5'-dithio-bis-2-nitro benzoic acid (DTNB), and resveratrol were purchased from the Sigma Chemical Company (USA).
Circular dichroism (CD) spectroscopy was conducted to determine the influence of the first sub-ambient temperature treatment on the secondary structure of β-lg. Far-UV CD spectra of 20 μM β-lg solutions (pH 9.5) at various temperatures (5, 10, 15, and 20°C) were measured three times using a 2 mm path length cuvette in a Chirascan CD spectrometer (Applied Photophysics, UK). To monitor the secondary structure of β-lg prepared at the first sub-ambient temperature treatment, scan wavelength range was from 195 to 260 nm with a 0.5-nm step resolution. CD spectra were averaged and normalized into units of mean residue ellipticity. Changes in the secondary structures of β-lg treated at the sub-ambient temperature from 5 to 20°C were analyzed using CD analysis software, CDNN (CD spectra Deconvolution Ver. 2.1; Gerald Böhm, Bioinformatics, University of Halle, Germany).
The effect of two-step temperature treatment on the surface hydrophobicity (S0) of β-lg was investigated using the modified method (Lee et al., 2013; Monahan et al., 1995). Hydrophobic fluorescent probe, ANS, was used to measure the surface hydrophobicity of β-lg. Thirty milligrams of β-lg were dissolved in 100 mL of 0.1 M Tris/HCl buffer (pH 9.5) and diluted to 0.005, 0.01, 0.015, 0.02, and 0.025% (w/v), respectively. For the first sub-ambient temperature treatment, 4 mL of diluted β-lg solutions was mixed with 20 μL of 8 mM ANS solution and kept at 5, 10, 15, or 20°C for 30 min. For the second mild heat temperature treatment, 4 mL of diluted β-lg solutions were heated at 55, 65, or 75°C for 10 min, cooled to room temperature, and mixed with 20 μL of 8 mM ANS solution. The fluorescent intensities of ANS solutions with and without β-lg solution were determined at an excitation wavelength of 390 nm and an emission wavelength of 470 nm using spectrofluorometer (Luminescence Spectrometer LS50 B, Perkin-Elmer Corp., USA) with 0.5 nm slit widths of both excitation and emission wavelengths. The initial slope of relative fluorescence (R) versus β-lg concentration (%, w/v) was used to express the surface hydrophobicity of β-lg and was calculated by linear regression analysis. The R value was calculated by following equation (Monahan et al., 1995).
R = (F − F0) / F0
where F is the fluorescent intensity of β-lg solution with ANS and F0 is the fluorescent intensity of ANS solution without β-lg.
The reactivity and accessibility of free thiol groups of β-lg treated with sub-ambient and mild heat temperature were determined by the modified method of Hoffmann and van Mil (1999). DTNB solution (0.133 g/g) was prepared by dissolving DTNB in 0.05 M Tris-HCl buffer (pH 9.5, containing 1 mM EDTA). For the first sub-ambient temperature treatment, 60 μL of β-lg solutions (1%, w/v) were kept at 5, 10, 15, or 20°C for 30 min and mixed with 2,340 μL of DTNB solutions. For the second mild heat temperature treatment, β-lg solutions were heated at 55, 65, or 75°C for 10 min, cooled to room temperature, and mixed with 2,340 μL of DTNB solutions. The absorbance at 412 nm was determined using a spectrophotometer. (GENESYS10-S, Thermo Inc., USA).
β-lg nanoparticles were prepared by the use of two-step temperature process. β-lg solutions (1%, w/v) were made by dissolving 0.1 g of β-lg powder in 10 mL of distilled water at room temperature. Forty microliter of 1 M CaCl2 solution was added to 10 mL of β-lg solution and then adjusted to pH 9.5±0.1 using 0.1 M NaOH. β-lg was treated at 5, 10, 15, or 20°C for 30 min (the first sub-ambient temperature treatment) followed by heating at 55, 65, or 75°C for 10 min (second mild heat temperature treatment). β-lg mixtures were changed from clear (transparent) to opaque suspension after the second mild heat treatment. After two-step heat treatments, β-lg suspensions were cooled to room temperature and used for further analysis.
Atomic force microscopy (AFM, XE-100, PSIA, Korea) was used to investigate the morphological characteristics of β-lg nanoparticles. Nanoparticle suspension was diluted with deionized water (1:10) and deposited on mica for 1 min. Unbound nanoparticles on mica were removed by washing with deionized water and air-dried at room temperature. AFM images of β-lg nanoparticles adsorbed on mica surface were observed in the non-contact mode of AFM with a resolution of 128 × 128 pixels and at a scan rate of 1 Hz. PPP-NCHR cantilever/tips (radius of curvature < 10 nm, Parks System) with a tip height of 125 μm and a force constant of 42 N/m, and a typical resonance frequency of 240 kHz were used. AFM images with a scan size of 2 μm2 were analyzed using XEI software (PSIA corp., version 1.5).
The physicochemical properties of β-lg nanoparticles including particle size and zeta-potential value were assessed using particle size analyzer (zetasizer Nano ZS, Malvern Instruments, UK). β-lg nanoparticle suspensions were 10-fold diluted with deionized water vortexed, and transferred into a 4-mL disposable polystyrene cuvette and a 1-mL clear zeta potential cuvette, respectively. The particle size of nanoparticles was assessed at room temperature using sing a laser beam at 633 nm and a scattering angle of 173°. The electrophoretic mobility (μm/s) of nanoparticle suspension was measured and converted into zeta-potential using the Smoluchowski equation.
All data were represented as the means ± standard errors. One-way analysis of variance (ANOVA) with the Fisher’s least significant difference (LSD) test was performed to investigate the impact of first sub-ambient temperature treatment and second mild heat temperature treatment on the secondary structure, surface hydrophobicity, and free thiol groups of β-lg and particle size and zeta-potential value of β-lg nanoparticles. The level of significance was set at 5% level (p<0.05). A significance in the effects of sub-ambient temperature, mild heat temperature, and their interactions on the size of β-lg nanoparticles was investigated by the use of two-way ANOVA. SAS software package (2003) was used to carry out all statistical analysis.
Results and Discussion
The structural changes of β-lg during two-step temperature process have been investigated with the analysis of CD, surface hydrophobicity, and free thiol groups (Hoffmann and van Mil, 1999; Monahan et al., 1995). Effect of sub-ambient temperature treatment on the conformational changes of β-lg was investigated using far-UV CD spectrometry (Fig. 1). As sub-ambient temperature was decreased from 20 to 5°C, a decrease in the negative ellipticity was observed while there were moderate shifts in the negative minima of CD spectra from 207 nm to 202 nm (Fig. 1(A)). It indicates that β-lg underwent conformational changes during sub-ambient temperature treatment. The changes of secondary structure of β-lg were exhibited in Fig. 1(B). A reduction in sub-ambient temperature from 20 to 5°C resulted in an increase in the amount of β-sheet and a decrease in the amount of a-helix (Fig. 1(B)). It implies that there was a transformation between a-helix and β-sheet secondary structures during sub-ambient temperature treatment. However, no changes in the amount of random coil of β-lg were observed (Fig. 1(B)). Native β-lg at neutral pH had about 58% of β-sheet content (Yang et al., 1986) while the amount of β-sheet in this study was about 23%, which is much lower than other studies. Similar results were reported in Taulier and Chalikian (2001). It may be due to alkaline denaturation of β-lg, which may lead to the loss of regular secondary structures (α-helix and β-sheet) and conversion to irregular structure (random coil).
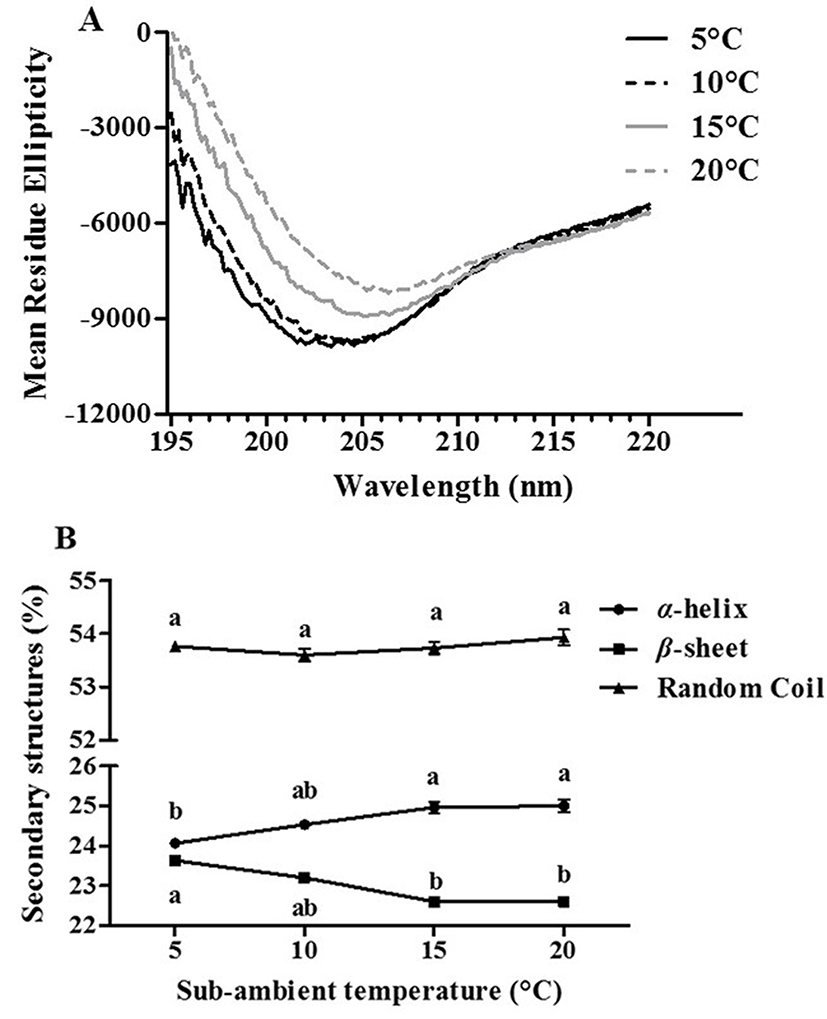
Impacts of the first sub-ambient temperature treatment on the surface hydrophobicity of β-lg were presented in Fig. 2(A). In this study, ANS, which is hydrophobic fluorescence probe, was used to determine the surface hydrophobicity of β-lg. Extrinsic aromatic hydrophobic probe, ANS, can bind the hydrophobic regions of proteins, which may result in an increase in the fluorescence intensity (Alizadeh-Pasdar and Li-Chan, 2000). A decrease in temperature from 20 to 5°C contributed to a significant (p<0.05) increase in the surface hydrophobicity of β-lg from 916 to 1,241 implying that there are more ANS molecules bound to the hydrophobic residues of β-lg at 5°C. In previous study, when β-lg prepared at pH 4.0 was incubated at below room temperature, an increase in the surface hydrophobicity of β-lg was observed due to the partial unfolding of β-lg (Ha et al., 2013). The partial unfolding of β-lg may lead to the exposure of hydrophobic amino acid groups present in inner proteins contributing to an increase in the fluorescence intensity. Therefore, lower sub-ambient temperature treatment at pH 9.5 can be an effective process to induce the partial unfolding of β-lg.
Effects of sub-ambient temperature treatment on the free, reactive thiol groups of β-lg were shown in Fig. 2(B). This method was based on reactions between DTNB and free, reactive thiol groups leading to form 1 mol of p-nitrothiophenol anion/mol of thiol (Hoffmann and van Mil, 1997). The formation of p-nitrothiophenol anion was assessed by measuring the absorbance at 412 nm. When sub-ambient temperature was decreased from 20 to 5°C, the absorbance was significantly (p<0.05) increased from 0.753 to 0.863 demonstrating that the accessibility of free, reactive thiol groups of β-lg with DTNB was enhanced at lower sub-ambient temperature (i.e., 5°C). The lower sub-ambient temperature treatment of β-lg could lead to the exposure of buried free, reactive thiol residues to the surface of β-lg, can promote the polymerization via thiol/disulfide exchanges (Hoffmann and van Mil, 1997).
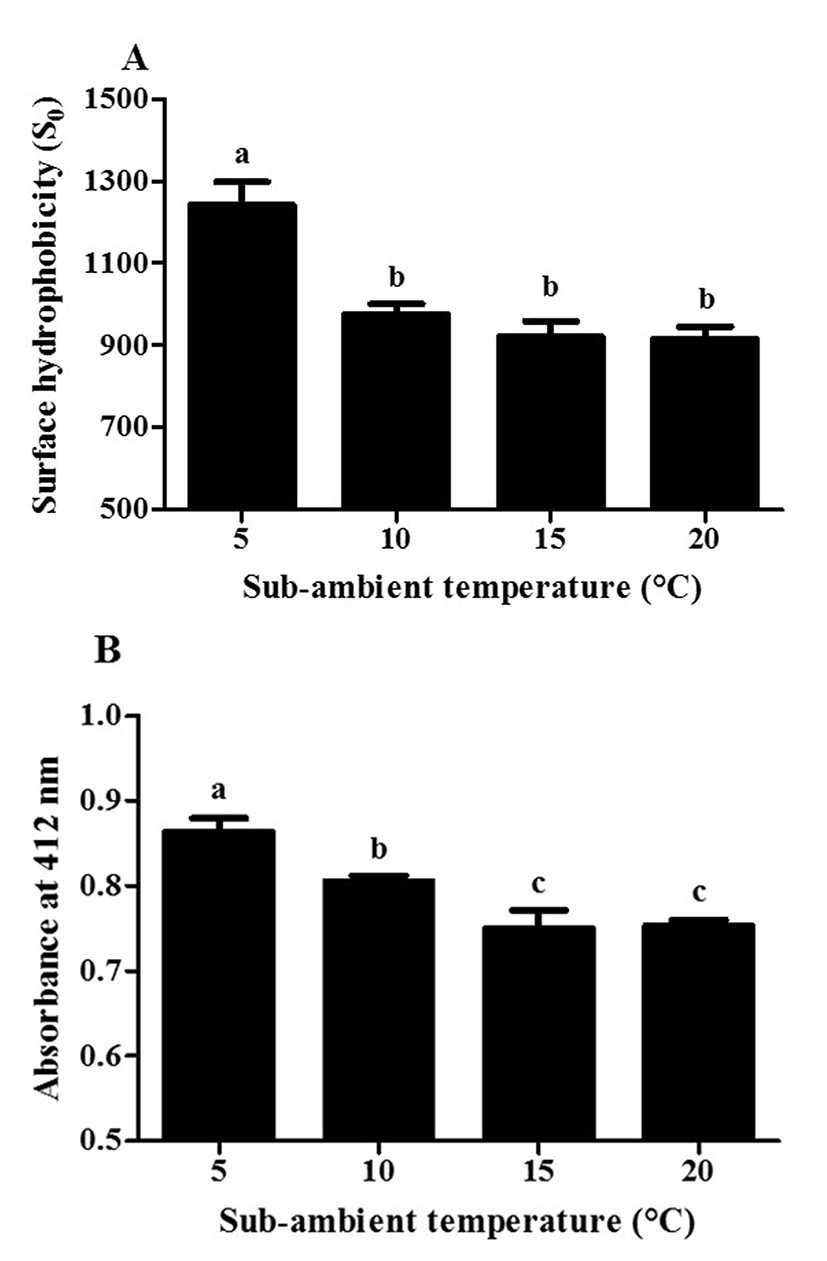
Fig. 3 shows the effects of the second mild heat temperature treatment on the surface hydrophobicity and free, reactive thiol groups of β-lg. There was a significant (p<0.05) increase in the surface hydrophobicity of β-lg from 929 to 1,585 with an increase in mild heat temperature from 55 to 75°C (Fig. 3(A)) demonstrating that the accessibility of ANS to the exposed hydrophobic residues of β-lg was enhanced at higher mild heat temperature (i.e., > 65°C). It has been reported that the reversible conformational changes of β-lg may occur at 40°C and further increase in temperature resulted in the formation of molten-globule-like structure, which can lead to the exposure of buried hydrophobic residues and free thiol groups of β-lg (Hoffmann and van Mil, 1997; Iametti et al., 1996). At neutral pH, β-lg undergoes irreversible conformational change in its tertiary structure including structural collapse into a molten-globule-like structure at above 65-70°C (Iametti et al., 1996; Prabakaran and Damodaran, 1997). Moreover, the denaturation temperature of β-lg was much lower at alkaline pH compared with neutral pH (Haug et al., 2009). These irreversible structural changes of β-lg could result in the rearrangement of β-lg molecules, which may lead to the polymerization of protein (Vetri and Militello, 2005). Our results suggest that during mild heat temperature process, the structural changes of β-lg leading to revealing of previously buried hydrophobic groups can be enhanced and mild heat temperature treatment can be an useful process to modulate the tertiary structure of β-lg. A similar result was reported that an increase in the surface hydrophobicity of whey protein isolate (WPI) at pH 9.0 was observed when heating temperature was increased from 55 to 75°C (Monahan et al., 1995).
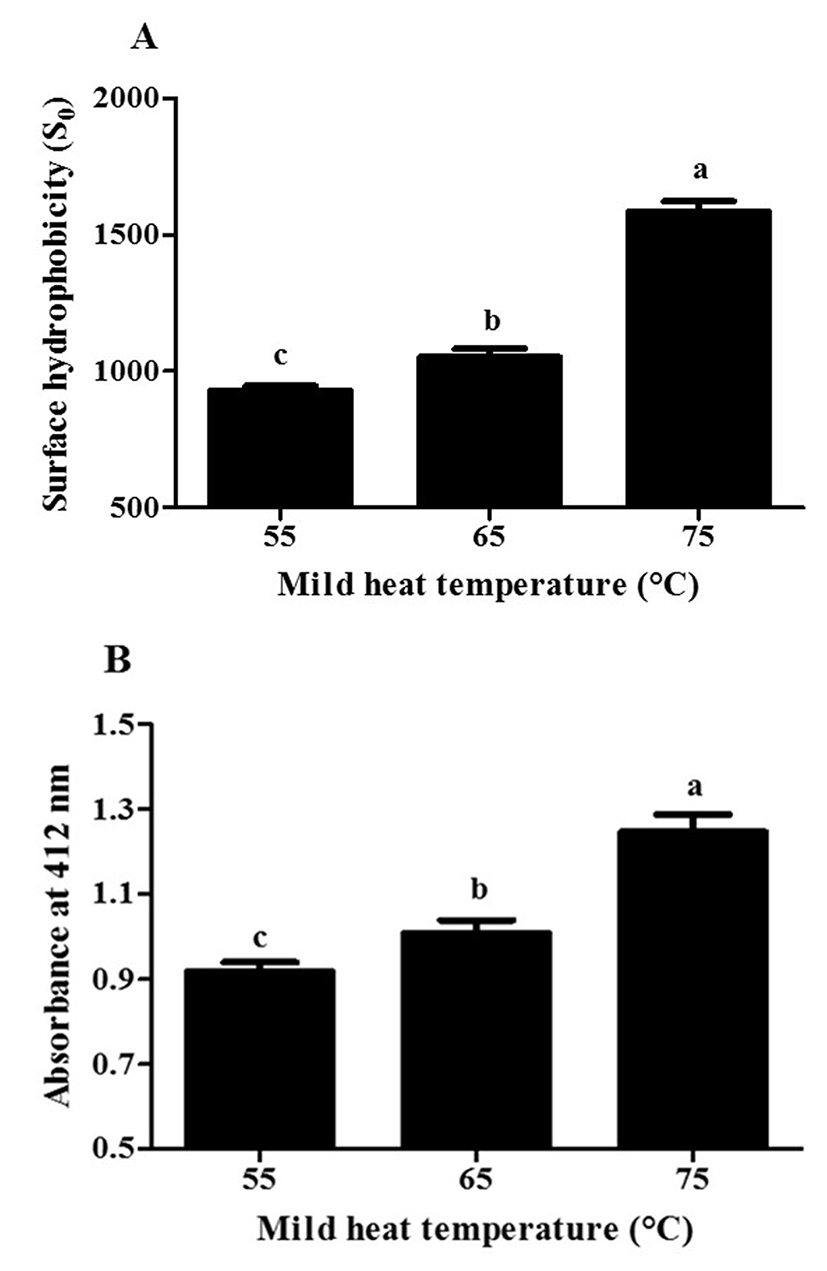
An increase in temperature from 55 to 75°C led to a significant (p<0.05) increase in free, reactive thiol groups of β-lg from 0.918 to 1.247 (Fig. 3(B)). It indicates that the exposure of free thiol groups of β-lg due to the conformational changes, such as the formation of molten-globule-like structure and/or denaturation of β-lg in its tertiary structure, can be increased with increased mild heat temperature (Iametti et al., 1996; Parris et al., 1991). An increase in the free, reactive thiol groups of whey proteins at alkaline pH (pH 9.0 and 11.0) was observed in 1% (w/w) WPI solution at pH 11 as temperature was increased from 50 to 75°C (Monahan et al. 1995).
In this study, the two-step temperature process with CaCl2 was used to manufacture β-lg nanoparticles at pH 9.5 (Ha et al., 2013; Leclerc et al., 2005). The schematic representation of production of β-lg nanoparticles was exhibited in Fig. 4(A). Since the use of calcium and increasing pH above the isoelectric pH of whey protein (i.e., ~5.3) can enhance electrostatic attractions between calcium and negatively charged groups of β-lg (Ha et al., 2015; Lee et al., 2013; Li et al., 1994), CaCl2 as a cross-linking agent was added to β-lg solutions followed by the adjustment of pH to 9.5. In Fig. 4(B), after the first sub-ambient temperature treatment from 5 to 20°C for 30 min, β-lg solutions remained transparent indicating that although the use of sub-ambient temperature treatment resulted in the development of reactive form of β-lg molecules with exposed free thiol groups as suggested in Fig. 2, β-lg underwent limited protein aggregations during this process. Opalescent β-lg solutions were observed when second mild heat temperature treatment was used (Fig. 4(B)) implying that more extensive aggregations in protein networks could be occurred and nanoparticles were developed. A degree of opalescence was increased as mild heat temperature was increased from 55 to 75°C (Fig. 4(B)). It demonstrates that much larger aggregates could be formed at higher mild heat temperature. Major driving forces for the heat-induced aggregation and gelation of whey proteins are thiol/disulfide exchange reactions and hydrophobic attractions (Hoffmann and van Mil, 1999). As seen in Fig. 3, higher surface hydrophobicity and accessible free thiol groups of β-lg treated at higher mild heat temperature (i.e., > 65°C) indicate that β-lg underwent conformational changes, which could lead to the production of more reactive forms of β-lg molecules. Moreover, an increase in temperature could enhance the strength of endothermic hydrophobic attractions between β-lg molecules, which may propagate their associations resulting in more extensive aggregations in protein networks (Bryant and McClements, 1998; Némethy and Scheraga, 1962; Zangi, 2011). Heat-induced aggregation of β-lg molecules at alkaline pH was studied by Monahan et al. (1995) using SDS-PAGE. They reported that the polymerization of whey proteins at pH 9.0 was enhanced when heat temperature was increased above 50°C.
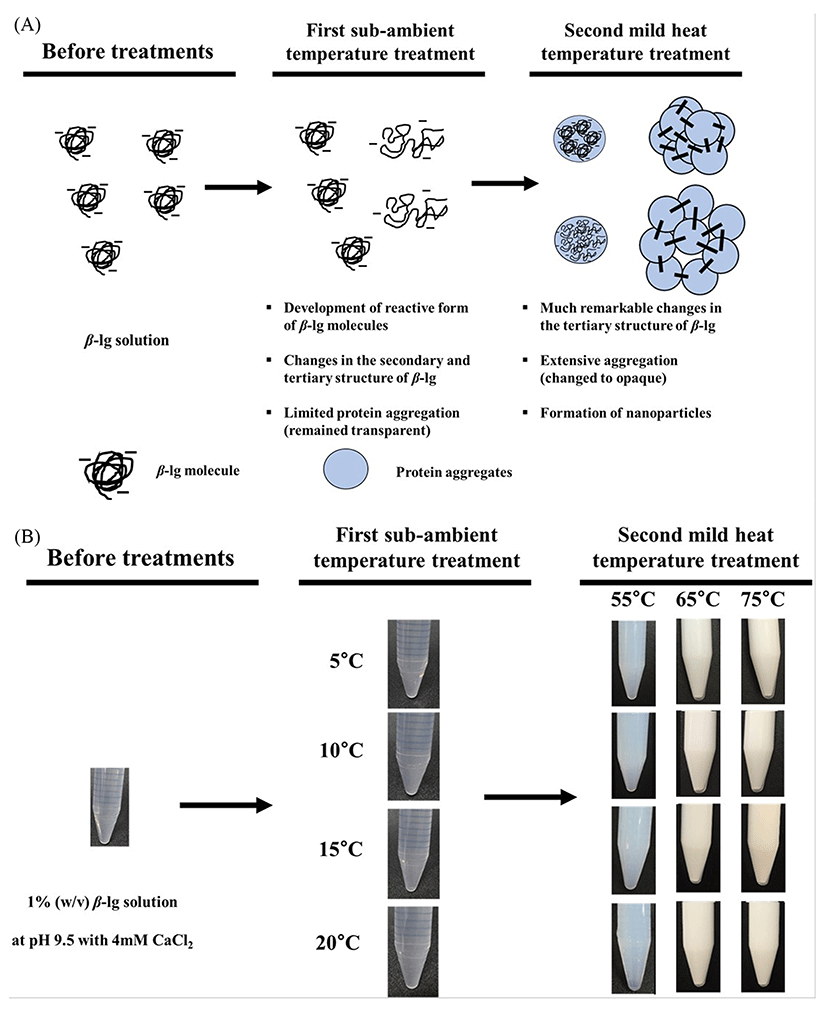
Impacts of the first sub-ambient temperature and second mild heat temperature treatments on the morphological properties of β-lg nanoparticles were shown in Figs. 5 and 6, respectively. In AFM images, spherical particles with a size ranging from about 100 to 200 nm were observed, which indicates that nanoparticles were successfully manufactured. Although the formation of nanoparticles would not be observed after the first sub-ambient temperature treatment without the second mild heat treatment as seen in Fig. 4(B), it seems that much larger nanoparticles were formed at lower sub-ambient temperature followed by the second mild heat temperature treatment at 75°C (Fig. 5). Moreover, an increase in mild heat temperature from 55 to 75°C resulted in the formation of larger nanoparticles (Fig. 6).
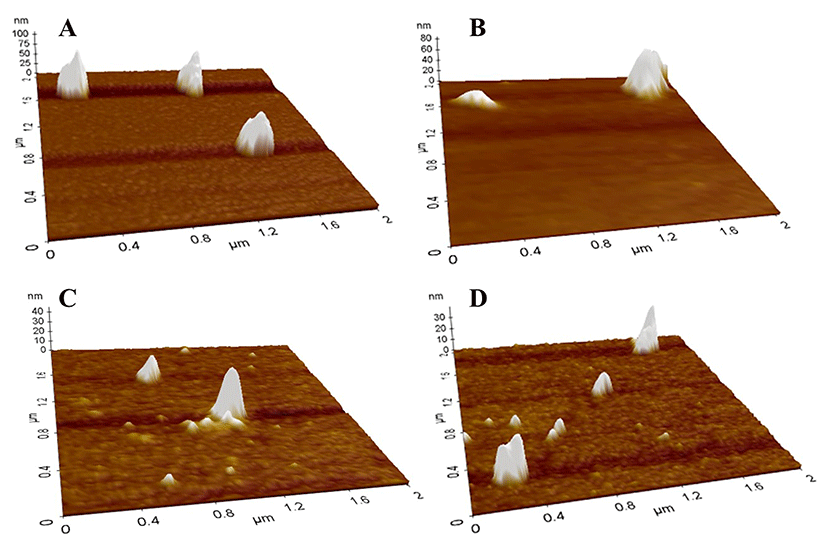
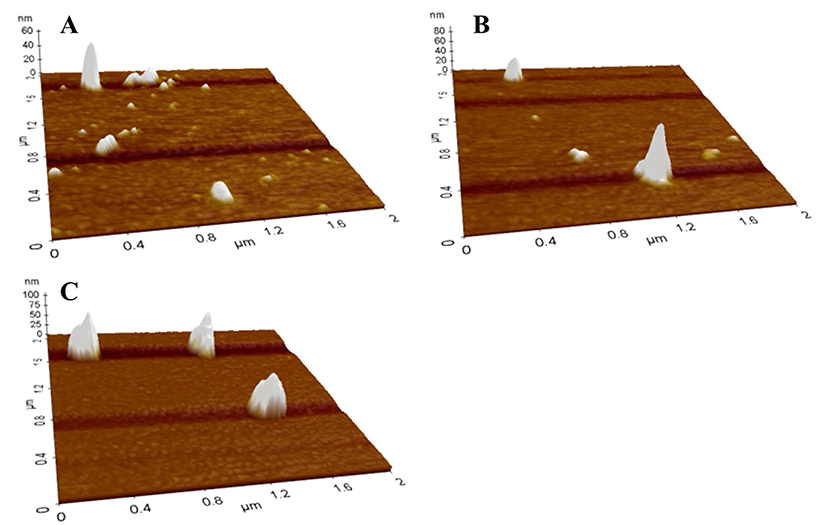
Effects of two-step temperature treatments on the physicochemical properties including particle size and zeta-potential value were exhibited in Figs. 7 and 8. Two-way ANOVA results presented that both the first sub-ambient temperature treatment and second mild heat temperature treatment significantly (p<0.0001) affected the size of β-lg nanoparticles (Fig. 7). As sub-ambient temperature was decreased from 20 to 5°C and mild heat temperature was increased from 55 to 75C, an increase in the particle size of β-lg nanoparticles was observed (Fig. 7). The use of lower sub-ambient temperature (i.e., 5°C) and higher mild-heat temperature treatment (i.e., 75°C) resulted in about 2-fold increase in the size of β-lg nanoparticles (106 to 214 nm) (Fig. 7) compared with higher sub-ambient temperature (i.e., 20°C). However, only 1.5-fold increase in the size of β-lg nanoparticles (61 to 94 nm) was observed when lower mild-heat temperature treatment (i.e., 55°C) was applied to β-lg solution treated at lower sub-ambient temperature (i.e., 5°C) compared with higher sub-ambient temperature (i.e., 20°C) (Fig. 7). It indicates that both sub-ambient and mild heat temperature treatment were critical to determine the size of β-lg nanoparticles during two-step temperature process. Although a similar transparency in β-lg solutions treated with various sub-ambient temperature from 5 to 20°C was observed in Fig. 4(B), critical changes in the secondary and tertiary structure of β-lg were occurred after 30 min of sub-ambient temperature treatment from 5 to 20°C (Figs. 1 and 2). An increase in β-sheet content at lower sub-ambient temperature (Fig. 1) demonstrates that the reactive form of β-lg molecules with exposed free thiol residues might be increased since the thiol groups of β-lg would be present in β-strand H (Prabakaran and Damodaran, 1997). Moreover, increases in the surface hydrophobicity and reactivity of free thiol groups of β-lg at lower sub-ambient temperature (Fig. 2) indicate that more hydrophobic and free thiol groups of β-lg were exposed. These results indicate that the use of sub-ambient temperature treatment may enhance the amount of reactive form of β-lg molecules with exposed free thiol groups and contribute to minor protein aggregation as suggested by a similar transparency in β-lg solutions.
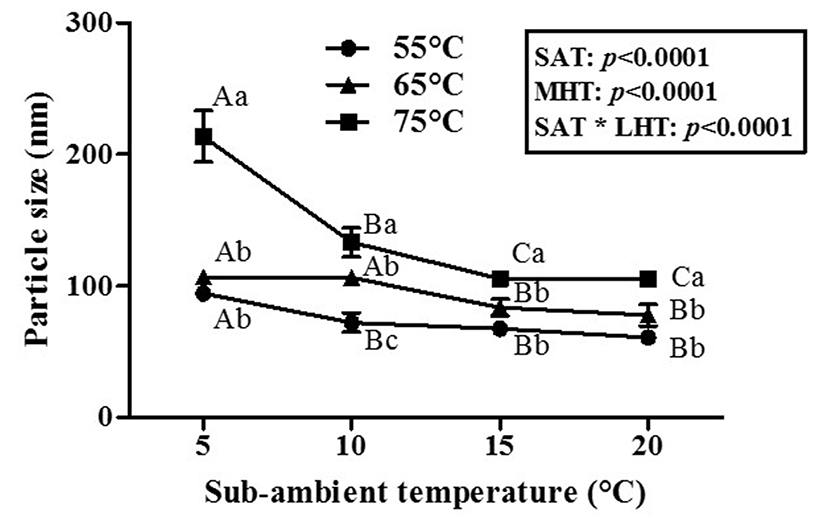
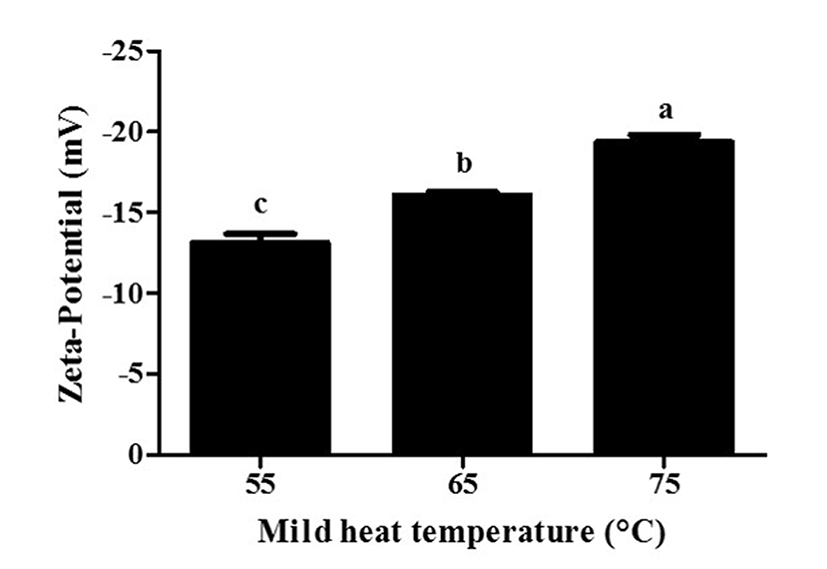
As seen in Fig. 7, higher mild heat temperature treatment led to a significant (p<0.05) increase in the size of β-lg nanoparticles treated at various sub-ambient temperature from 5 to 20°C. Changes in the tertiary structure of β-lg treated with the second mild heat temperature for 10 min were much remarkable (surface hydrophobicity from 0.929 to 1,585 and reactivity of free thiol groups from 0.918 to 1.247), than those of the first sub-ambient temperature treatment (surface hydrophobicity from 916 to 1,241 and reactivity of thiol groups from 0.753 to 0.863) (Figs. 2 and 3). Unfolded hydrophobic and thiol residues of β-lg could enhance protein polymerization and aggregation via hydrophobic interactions and thioldisulfide exchanges (Ha et al., 2015; Monahan et al., 1995). Since endothermic hydrophobic attractions and intermolecular disulfide bonds have been known as major attractive forces between whey protein molecules, an increase in mild-heat temperature from 55 to 75°C could enhance intermolecular associations between β-lg molecules, which may contribute to an increase in the size of β-lg nanoparticles (Gracia-Julia et al., 2008; Hoffmann and van Mil., 1999; Li et al., 1994). In Fig. 4(B), noticeable changes in the opaqueness of β-lg solution were observed after mild heat temperature treatment at 65 and 75°C. These results imply that higher mild heat temperature treatment at > 65°C is a critical step to induce more reactive form of β-lg molecules and extensively propagate the aggregate reactions of β-lg compared with sub-ambient temperature treatment, which can lead to an increase in the size of β-lg nanoparticles.
β-lg nanoparticles had negative surface charges (Fig. 8). A decrease in the zeta-potential value of β-lg nanoparticles from -13.1 to -19.3 mV was observed with an increase in mild heat temperature from 55 to 75°C (Fig. 8) while sub-ambient temperature treatment did not affect the zeta-potential values of β-lg nanoparticles. A reduction in the zeta-potential value at higher mild heat temperature indicates an increase in the exposure of previously buried negatively charged amino acids present in β-lg (Ha et al., 2015).
Conclusions
In conclusion, β-lg nanoparticles with a size ranging from 61 to 214 nm were successfully manufactured using two-step temperature process that is the first sub-ambient temperature treatment followed by second mild heat treatment. During two-step temperature process, both the first sub-ambient temperature and second mild heat temperature treatment were critical factors in inducing the conformational changes of β-lg, such as secondary structure changes in α-helix and β-sheet and tertiary structure changes in the exposure of previously buried hydrophobic and free thiol groups, and determining the physicochemical properties of β-lg nanoparticles, such as particle size and surface charge. Two-step temperature process can be used as a simple, but effective manufacturing process to modulate the physicochemical properties of β-lg nanoparticles.