Introduction
Numerous studies have provided evidence demonstrating the impact of lactic acid bacteria (LAB) on the equilibrium of the host’s microbiota, and their ability to modulate host immunity and facilitate several advantageous physiological processes (Kim et al., 2020; Markowiak and Śliżewska, 2017). Thus, the hydrophobicity, adhesion, and colonization capacity of cell surfaces are crucial characteristics (Ouwehand et al., 2002), as these attributes serve as the foundation for facilitating the probiotics’ enduring colonization of the host’s intestinal tract and enable the manifestation of their physiological functionalities (Collins et al., 1998).
LAB are exposed to a variety of stressors from the external environment during fermentation, processing, and storage within the gastrointestinal tract of the host. LAB often encounter temperature fluctuations, which is the prevalent environmental stressor. Moreover, LAB frequently encounter weakly acidic and alkaline conditions, which serve as stressors during fermentation and also during the bacteria’s transit through the large and small intestines (Liu et al., 2007). Sodium chloride (NaCl), or salt, is a fundamental food additive due to its ability to enhance flavor and taste attributes and extend the shelf life of food products. However, the introduction of elevated levels of salt into dairy products, such as cheese and butter, has the potential to impact the efficacy of probiotics and elevate the host’s susceptibility to various diseases (Wang et al., 2016).
LAB can generate adaptive responses to environmental stressors and possess a multitude of self-regulatory mechanisms. For example, research findings indicate that probiotics respond to intricate environmental pressures through alterations in the composition of fatty acids inside their cell membranes; this adaptive process serves as a defensive mechanism (Bevilacqua et al., 2010). Furthermore, the ability of LAB to resist osmotic pressure is influenced by the ratio of saturated to unsaturated fatty acids in their cell membranes, as well as the process of cyclopropanation involving oleic acid (Gong et al., 2012; Montanari et al., 2010). Escherichia coli is a prevalent bacterium associated with foodborne illnesses. LAB can impede the attachment and establishment of harmful bacteria, such as E. coli, in the gastrointestinal tract, either by employing auto-aggregation or forming aggregates with the pathogens (García-Cayuela et al., 2014). Therefore, E. coli was selected for the co-aggregation experiments in this study.
The growth, metabolism, and physiological activities of LAB can be affected, to varing degree, by different growth environment stressors and the fluidity of their membranes (Guillot et al., 2000). Furthermore, the composition of fatty acids produced during fermentation may vary and influence the bacteria’s hydrophobic characteristics and adhesive capacities. In this study, we investigated the cell surface characteristics of JNU532 under different environmental stresses, including hydrophobicity, auto-aggregation and co-aggregation abilities, and adhesion to HT-29 intestinal cells. Additionally, we analyzed changes in the fatty acid composition of JNU532 cells, hypothesizing that these changes might influence its cell surface properties.
Materials and Methods
The Limosilactobacillus fermentum JNU532 used in this study was isolated and screened from kimchi in a previous study (Kim et al., 2023). L. fermentum JNU532 was cultivated in de Man, Rogosa, and Sharpe (MRS) medium (1 L: glucose 20 g, peptone 10 g, beef extract 10 g, yeast extract 5 g, sodium acetate 5 g, magnesium sulfate 0.2 g, manganese sulfate 0.05 g, dipotassium phosphate 2 g, ammonium citrate 2 g, and tween 80 1 g) for 24 h at 37°C.
The L. fermentum JNU532 (100 μL; 109 colony forming units CFU/mL) was added to MRS medium at pH 4 (acid), 6.5, and 8 (alkaline) and MRS medium+3% NaCl and incubated at 37°C for 24 h. In addition, MRS medium (pH 6.5) inoculated with L. fermentum JNU532 was incubated at 42°C for 24 h (Table 1). The MRS medium’s pH was adjusted using 5N HCl and 5N NaOH to produce the various pH levels. Cell was counted was achieved using the standard plate count method. The isolated L. fermentum JNU532 was serially diluted in anaerobic diluent and cultured in MRS medium for 24 h before counting. The survival rate of L. fermentum JNU532 was used for the cell adhesion experiments.
Stress | Temperature (°C) | pH | Time (h) |
---|---|---|---|
Control | 37 | 6.5 | 24 |
Temperature | 42 | 6.5 | 24 |
3% NaCl stress | 37 | 6.5 | 24 |
Acid stress | 37 | 4 | 24 |
Alkaline stress | 37 | 8 | 24 |
Auto-aggregation ability of JNU532 was determined by the method of Collado et al. (2008) with slight modifications. The L. fermentum JNU532 subjected to the different stress treatments were incubated at 37°C for 24 h. Then, the culture solution was centrifuged at 2,000×g for 15 min at 4°C and the recovered pellet was washed twice with phosphate-buffered saline (PBS) at pH 7.2. After that, the supernatant was removed, and the cell pellet was resuspended in PBS to adjust the bacterial suspension to an absorbance of 1.0 at 600 nm, this was recorded as A0. Then, 1 mL of the bacterial suspension was allowed to stand at 37°C for 2 h before the absorbance of the supernatant suspension (A2) was measured at 600 nm was measured using a microplate reader (Synergy HTX, BioTek, Shoreline, WA, USA). The auto-aggregation ability was calculated using the following formula:
The co-aggregation ability was determined refer to previous research (Golowczyc et al., 2007) with slight modifications. The L. fermentum JNU532 and pathogenic bacterium E. coli suspensions subjected to different stress treatments were prepared according to the method described for the auto-aggregation ability assay. The absorbance values of the E. coli and L. fermentum JNU532 suspensions at 600 nm were designated as Apath and Aprobio, respectively. In addition, the L. fermentum JNU532 suspensions (2 mL) subjected to different stress treatments were mixed with an equal volume of E. coli suspension (2 mL) and cultured at 37°C for 2 h. The absorbance was measured at 600 nm was measured using a microplate reader (Synergy HTX, BioTek) and recorded as Amix. The co-aggregation ability was calculated using the following formula:
The surface hydrophobicity was determined by the adhesion of bacteria to hydrocarbons using a slightly modified xylene extraction method (Rosenberg et al., 1980). The L. fermentum JNU532 were cultured at 37°C for 18 h in MRS broth and then harvested by centrifugation (3,000×g, 10 min, 4°C) and washed and resuspended in phosphate urea magnesium buffer (PUM; K2HPO4; 17 mg/mL), potassium dihydrogen phosphate (KH2PO4; 7.26 mg/mL), urea [CO(NH2)2; 1.8 mg/mL], and magnesium sulfate heptahydrate (MgSO4.7H2O; 0.2 mg/mL) to an absorbance of 1.0 at 600 nm was measured using a microplate reader, this was recorded as A0. The bacterial suspension (2 mL) was added to an equal volume of xylene and the two-phase system was vortexed for 2 min for mixing and allowed to stand at room temperature for 1 h. Then, the aqueous phase was removed, and its absorbance was measured at 600 nm was measured using a microplate reader, this was recorded as A. Surface relative hydrophobicity was calculated by the following formula:
The HT-29 cells (KCLB 30038), human colorectal cancer cells, were obtained from the Korean Cell Line Bank (Seoul, Korea), derived from human colon adenocarcinoma, was cultured using standard procedures. Dulbecco’s modified Eagle medium (DMEM, Welgene, Namcheon, Korea) was used as the growth medium, supplemented with 10% fetal bovine serum (Corning, Glendale, AZ, USA), 100 U/mL penicillin, and 100 μg/mL streptomycin (Welgene). The cells were maintained in a CO2 incubator (PHCbi, Tokyo, Japan) at a temperature of 37°C, in a humidified environment consisting of 5% CO2 and 95% air. On reaching 80% confluence, the cells underwent digestion using a solution of 0.25% trypsin-ethylenediaminetetraacetic acid (EDTA; Welgene), followed by subculturing in DMEM. To ensure the viability of the cells, the culture media was replaced every 48 h.
The adhesion experiment employed second-generation sub-cultured HT-29 cells. The HT-29 cells were obtained and cultivated on 6-well culture plates at a temperature of 37°C in a humid environment with 5% CO2. The initial cell density in each well was 5×105 cells. The cultures were cultivated until they reached a coverage of 85%–95% of the surface area. The media was fully replaced with a new DMEM medium devoid of antibiotics 24 h before the adhesion experiment. Before the experiment, the cells underwent two rounds of washing with PBS (pH 7.2). The suspensions of L. fermentum JNU532 cultivated under various stress conditions were reconstituted for 24 h in DMEM without antibiotics and fetal bovine serum to a density of 1×107 CFU/mL. The reconstituted suspension was incubated for 2 h at 37°C in a 5% CO2 environment.
Non-adhering bacteria were removed from the HT-29 cells by rinsing them three times with PBS buffer. To release attached bacterial cells, the HT-29 cell cultures were treated with a solution of 1% Triton X-100 (Sigma-Aldrich®, Darmstadt, Germany). The lysis was performed on ice for 10 min. The lysates were centrifuged at 2,000×g for 10 min and the precipitate was washed twice with PBS. Finally, the precipitate was suspended in 1 mL of anaerobic diluent and the number of adhered bacteria was quantified according to the serial dilution method. Serial decimal dilutions ranging from 10–1 to 10–5 CFU/mL was prepared and plated out on a solid MRS medium. After the incubation of plates with 5% CO2 at 37°C for 24 h, the number of L. fermentum JNU532 colonies was counted. Three independent experiments were conducted, and the results were statistically evaluated.
The cellular fatty acid content of the isolated L. fermentum JNU532 was analyzed using Miller’s method (Miller, 1982; Park et al., 2010; Yang et al., 1993). Following the transfer of about 40 mg of grown cells into a Teflon-lined screw cap tube, 1 mL of a solution comprising 15% NaOH in 50% methanol was introduced. Subsequently, the mixture was heated to 100°C for 30 min, after which it was allowed to cool down to room temperature. Then 2 mL of a methanolic solution of hydrochloric acid (HCl), a mixture consisting of 325 mL of 6.0 N HCl and 275 mL of methanol, was added. The resulting mixture was heated to 80°C for 10 min. Subsequently, the mixture was rapidly cooled, and 1.25 mL of a solution containing hexane and methyl-tert-butyl ether (1:1, v/v) was added; the mixture was mixed well for 10 min. The reaction solution experienced phase separation on cooling to room temperature, resulting in the formation of two distinct layers. Subsequently, the lower liquid layer was extracted. Following this, 3 mL of diluted sodium hydroxide (NaOH) solution with a concentration of 10.8 g NaOH per 900 mL of deionized water was added to the system. The mixture was thoroughly agitated for 10 min and subsequently allowed to stand at room temperature, facilitating the dissolution of about two-thirds of the supernatant. The sample was put into a screw-capped sample vial (12×32 mm, Agilent Technologies, Santa Clara, CA, USA) and securely secured for further analysis.
The Agilent 6890N Network Gas Chromatograph (Agilent Technologies). The produced sample was subjected to gas chromatography analysis, utilizing a 30 m×0.320 mm×0.25 μm crosslinked methyl siloxane column (HP-1, Agilent Technologies) for the separation process. The analysis utilized the MIDI Sherlock™ Microbial Identification System (MIS) software (v. 6.3, Agilent Technologies). In contrast to the conventional calibration solution, the following parameters were used: peak identification, retention duration, peak area, and area ratio.
Results were subjected to a one-way analysis of variance (ANOVA) by Duncan’s multiple range test and are presented as the mean±SE. The analysis was performed using SPSS® (v. 26.0 for Windows, IBM Statistical SoftwareTM, IBM, Armonk, NY, USA). Significant difference was determined at values of p<0.05 (*), p<0.01 (**), and p<0.001 (***), respectively.
Results
Fig. 1 shows the changes in the growth of JNU532 during a 24-h culture period under various environmental stress conditions. The strain was modestly hindered by an elevated temperature of 42°C. In comparison to the control group (pH 6.5) maintained at a temperature of 37°C, there was no significant difference in the survival rate of the bacteria subjected to acidic stress (pH 4) and the alkaline (pH 8) stress group.
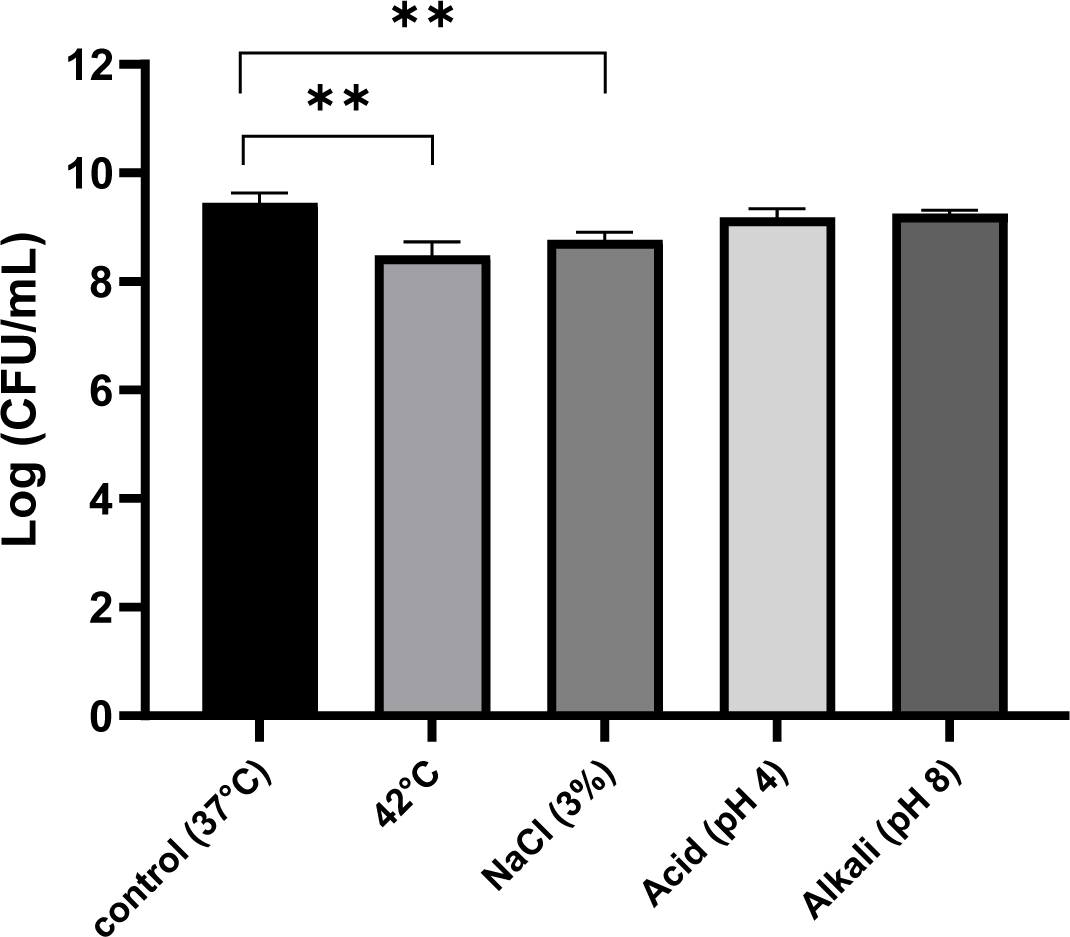
The viability of LAB isolated from table olives was investigated in order to determine the effects of synthetic gastric and pancreatic fluids. It was found that the survival rate of LAB to pancreatic juice digestion was superior compared to that observed for gastric juice (Bautista-Gallego et al., 2013). In this study, the resistance of JNU532 to acid was shown to be comparatively low compared with its resistance to alkali stress. The addition of a low concentration of salt (3%) to the MRS medium resulted in a small inhibition in the growth of JNU532 in comparison with the control.
Auto-aggregation of JNU532 was significantly increased at elevated temperature, reaching a value of 16.73%, which was markedly greater compared with the control group’s value of 7.39%. There was no notable disparity between the stress group cultured in MRS medium at pH 8 (7.41%) and the control group (7.39%). Compared to the control group, the stress groups cultured at a pH 4 (9.13%) and with 3% NaCl (11.74%) demonstrated that environmental stress did not have a detrimental effect on the auto-aggregation capacity of JNU532; rather, it appeared to enhance the strain’s ability to auto-aggregate.
Table 2 shows the co-aggregation capability of L. fermentum JNU532 with E. coli, as well as its auto-aggregation capability. The temperature stress group exhibited auto-aggregation and co-aggregation capacities of 16.73±0.15% and 82.85±0.01%, respectively, compared with the control. When JNU532 was exposed to low-concentration osmotic, acid, and alkaline stresses exhibited enhanced auto- and co-aggregation abilities compared with the control group. In contrast, the control group had the lowest auto- and co-aggregation abilities at 7.39±0.51% and 77.96±0.32%, respectively.
Auto-aggregation | Co-aggregation (Escherichia coli) | |
---|---|---|
37°C | 7.39±0.51 | 77.96±0.32 |
42°C | 16.73±0.15*** | 82.85±0.01** |
3%NaCl | 11.74±0.67** | 78.23±1.15 |
pH 4 | 9.13±0.42** | 77.47±0.82 |
pH 8 | 7.41±0.46 | 76.48±0.50* |
Control: Man, Rogosa, and Sharpe (MRS) medium (pH 6.5), at 37°C; 42°C: MRS medium (pH 6.5), at 42°C; 3% NaCl: MRS medium+3% NaCl, at 37°C; pH 4: MRS mediums (pH 4), at 37°C; pH 8: MRS mediums (pH 8), at 37°C for 24 h.
Cellular hydrophobicity are crucial for the adherence of probiotic bacteria to the cells lining the intestines, facilitating their establishment in the gastrointestinal system and enhancing their beneficial physiological impact. Research has demonstrated that strains with higher hydrophobicity exhibit increased adherence. The surface hydrophobicity of LAB is highly associated with adhesion capability of bacterial strains (Zago et al., 2011). Fig. 2 demonstrates the adhesive capacity of JNU532 to HT-29 cells. When L. fermentum JNU532 was cultivated under acid stress, it exhibited the highest adhesion ability at 91.55%. The alkaline stress group’s adhesion ability (81.4%) was compared with that of the control group (85.94%); the adherence was feeble and the adhesion capacity was somewhat hindered. Fig. 3 shows that the surface hydrophobicity of JNU532 cells subjected to alkaline stress was greater than the control group; however, the adhesion capability was inferior compared with the control group. From the results of hydrophobicity and adhesion under alkaline stress, there seems to be no direct correlation between surface hydrophobicity and adhesion capability. This is inconsistent with research findings that show a highly associated between hydrophobicity and adhesion capability. However, it aligns with previous research suggesting that alkaline stress may cause damage to the cell membrane, thereby reducing adhesion capability (Wang et al., 2018). Moreover, exposing JNU532 to a mild osmotic stress (3% NaCl) resulted in an enhancement in its adhesion capacity (78.21%) compared with the control group.
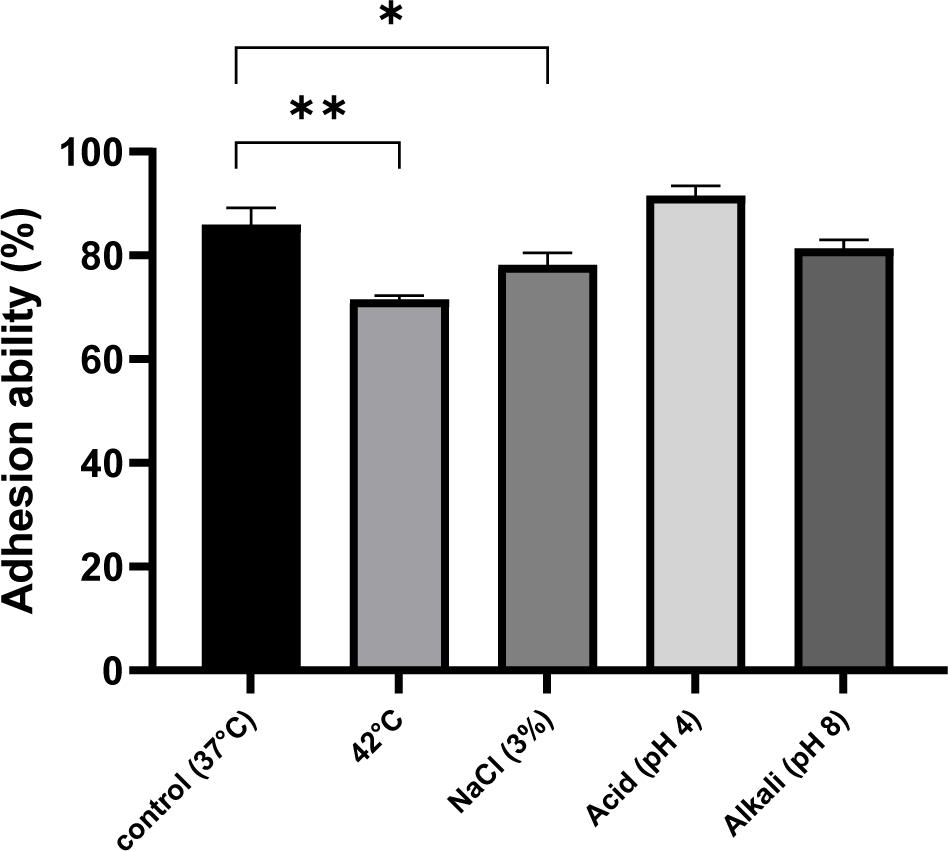
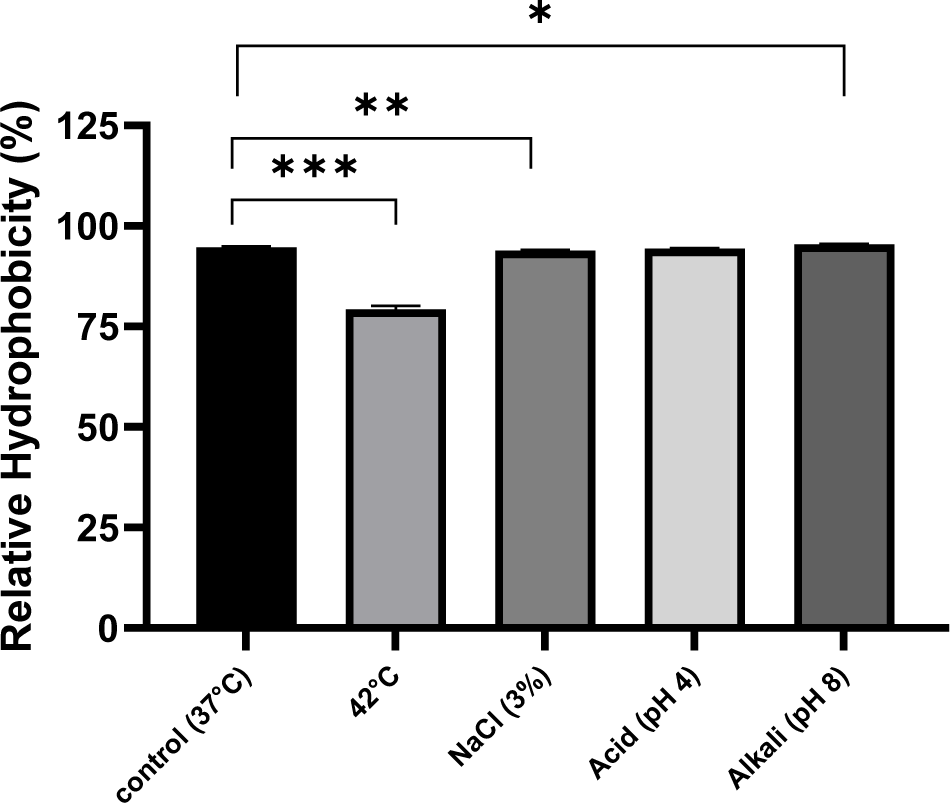
Table 3 reports the fatty acid constituents of JNU532’s cell membranes; the control, osmotic, and acid stress groups exhibited little alterations in fatty acid composition (Fig. 4). The primary fatty acid components in the control group’s cell membranes were hexadecanoic acid (C16:0: 32.06%), oleic acid (C18:1: ɷ9c 20.07%), cis-9,10-methyleneoctadecanoic acid (C19:0 cyclo ɷ8c:12.28%), and C19:0 cyclo ɷ10c/19ɷ6 (9.35%). JNU532, when subjected to a temperature of 42°C and alkaline stress, exhibited higher levels of Icosanoic acid (C20:0) in the cell membrane, with percentages of 17.03% and 23.41%, respectively. At a temperature of 42°C, the proportion of C16:0 (21.9%) decreased, while the proportion of C18:1 ɷ9c (22.75%) increased. Notably, the proportion of C19:0 cyclo ɷ10c/19ɷ6 (17.66%) increased dramatically compared with control.
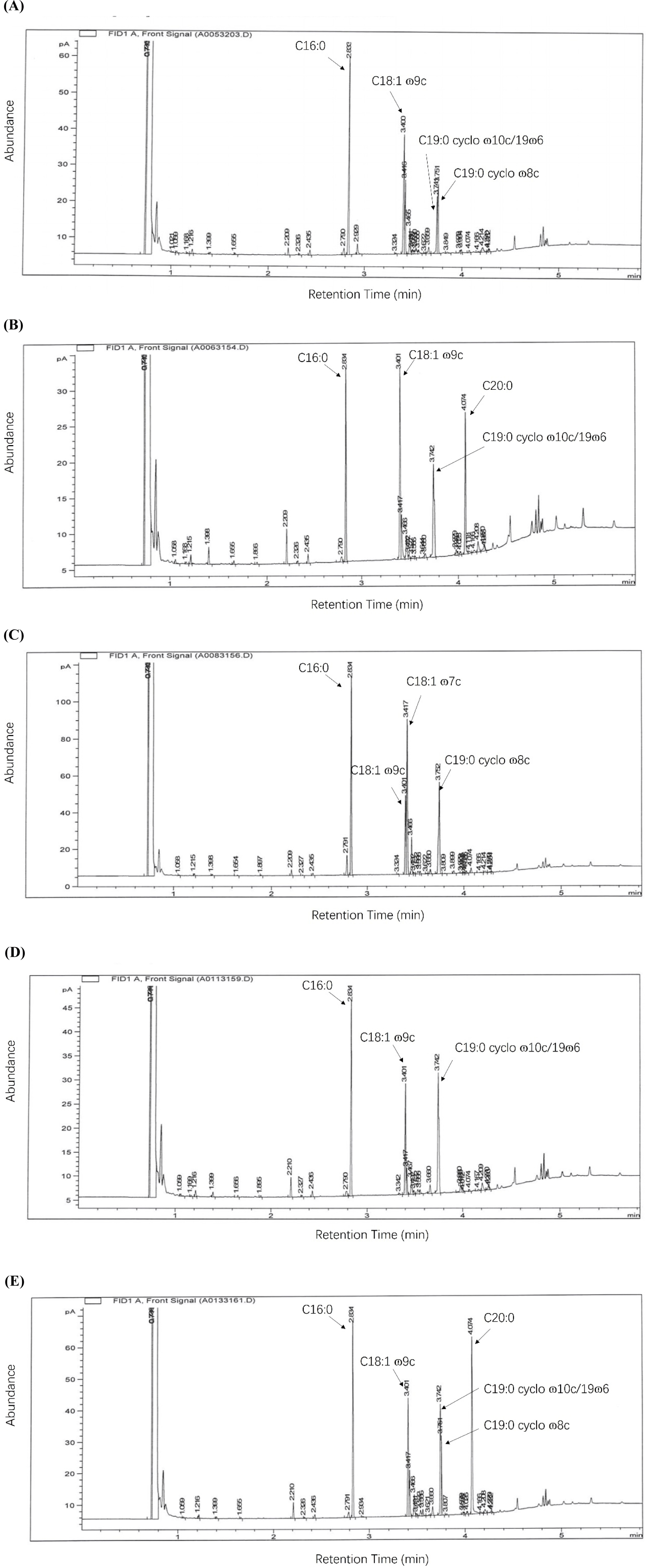
When L. fermentum JNU532 was exposed to mild osmotic stress, the cell membrane content of C16:0 (29.51%) and C18:1 ɷ9c (12.26%) declined, whereas the content of C19:0 cyclo ɷ8c (19.13%) increase compared with the control group. When JNU532 was subjected to acidic stress, the cell membranes’ content of C16:0 (31.83%) and C18:1 ɷ9c (18.02%) did not vary significantly compared with the control group but showed a modest decrease. In contrast, the cell membranes’ concentration of C19:0 cyclo ɷ10c/19ɷ6 exhibited a substantial rise, reaching 30.88%, which was the highest among all the experimental groups. The primary fatty acid constituents of JNU532’s cell membranes in the presence of alkaline stress were C16:0 (23.41%), C18:1 ɷ9c (14.43%), C19:0 cyclo ɷ8c (8.09%), and C19:0 cyclo ɷ10c/19ɷ6 (14.67%).
Discussion
A previous study found that the fermentation broth of JNU532 had antioxidant properties and could reduce melanin production by inhibiting the activity of tyrosinase in B16F10 melanoma cells. Therefore, JNU532 could be a valuable natural depigmentation agent (Meng and Oh, 2021). The results of the present study indicate that JNU532 exhibited specific defense mechanisms against various environmental stress including auto- and co-aggregation, alterations in cellular fatty acid composition, and cell surface hydrophobicity. On exposure to acidic conditions, JNU532 exhibited a similar growth rate and surface hydrophobicity superior to that in control group; however, its adhesion ability was somewhat superior to that in control group. Increased adhesion capacity on exposure to acidic conditions may be explained by JNU532 cell membranes’ increased proportion of C19:0 cyclo ɷ10c/19ɷ6 compared with that in the cell membranes in other stress groups.
The fatty acid composition analysis revealed that the C18:1 ɷ9c ratio in the cell membranes of the 3% NaCl stress group was 12.16%, which was the lowest ratio compared with the other stress groups. This result suggests that NaCl is responsible for the decreased C18:1 ɷ9c ratio; in contrast, it increased the C19:0 cyclo ɷ8c ratio to 19.13%.
During conditions of heat stress compared with other stress, there was a drop in the L. fermentum JNU532 cell membranes’ ratio of C16:0 and an increase in C18:1 ɷ9c; the smallest ratio was seen for C19:0 cyclo, this is considered to be one factor that influences the surface characteristics of the cell membrane. The content of unsaturated long-chain fatty acids increases in the JNU532 cell membrane when the surrounding environment changes is to regulate the fluidity and flexibility of the cell membrane. Unsaturated fatty acids contain double bonds, which significantly impact the structural properties of the cell membrane (Streit et al., 2008).
A 5°C increase in the environment’s temperature, constituting mild heat stress, results in improved acid production. This change causes the cell membrane to become less fluid and more rigid, which can restrict its flexibility and potentially harm the cell. Unsaturated fatty acids, characterized by their double bonds, enhance the cell membrane’s flexibility. This allows the membrane to adapt more effectively to environmental changes. Additionally, these unsaturated fatty acids improve the fluidity of the cell membrane, helping to maintain its structural integrity. Furthermore, the bacterial count was assessed at three-hour intervals spanning from 0 to 24 h. The findings indicated that the number of JNU532 decreased under mild heat stress conditions, commencing at the 18-h mark. Glucose could undergo denaturation when exposed to sustained mild heat stress, leading to an impact on the growth of the strain (Fig. 5). Additionally, mild heat stress decreased the hydrophobicity of the strain surface and impaired its capacity to adhere to cells. Nevertheless, heat stress enhanced the auto- and co-aggregation capabilities of JNU532. In addition, prior research has failed to find relationship between hydrophobicity and adhesion capability (Haddaji et al., 2015; Vinderola et al., 2004). However, several research indicate results revealed a link between cell hydrophobicity and adhesion. Reports indicate a significant increase in the production of proteins associated with the adhesive capacity of LAB in response to acidic stress (Ma et al., 2020; Wang et al., 2018). In addition, these authors suggested that the decline in adhesive capacity under alkaline stress was associated with several mechanisms that regulate pH (Ma et al., 2020). In this study, all experimental groups, except for the alkaline stress group, showed that the adhesion capacity of JNU532 to HT-29 cells increased when the bacteria’s surface exhibited a high degree of hydrophobicity. The one-way ANOVA results indicated that JNU532 subjected to alkaline stress exhibited significant surface hydrophobicity but reduced adhesion capability on HT-29 cells; these results are consistent with a prior study’s findings (Ma et al., 2020).
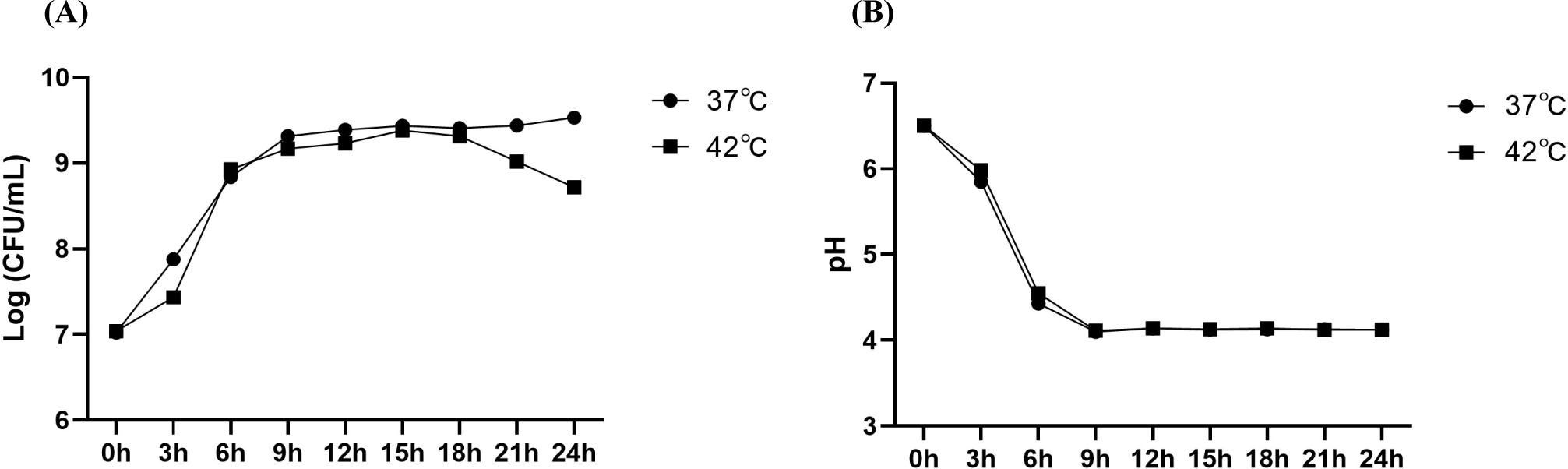
Previous studies have established a correlation between the ratio of unsaturated and saturated fatty acids and cell membrane fluidity (Casadei et al., 2002; Vesterlund et al., 2005). Cells attain a level of fluidity that is compatible with the host by modifying the composition of their lipids (Teixeira et al., 2002). For example, Listeria innocua may modify the content of fatty acids on its cell surface when exposed to different environmental conditions, such as cold, heat, acid, and starvation. Alterations in fatty acid content can also influence membrane fluidity and cell hydrophobicity. In addition, membrane fatty acids can alter branching length and saturation in response to environmental stress, which modifies membrane fluidity and the cell’s interaction with its surroundings (Lagha et al., 2012; Moorman et al., 2008). This study observed variations in the branch chain length and saturation of the stresses at different pressures. Under heat stress conditions, JNU532 produced 3-hydroxy 15-methylhexadecanoic acid (C17:0 iso 3OH: 0.69%) and paullinic acid (C20:1 ɷ7c: 0.47%). The 11-eicosenoic acid (C20:1 ɷ9c) and C20:1 ɷ7c yields were 0.09% and 0.37%, respectively, when L. fermentum JNU532 was subjected to NaCl concentrations lower than 3%. Similarly, when L. fermentum JNU532 was exposed to acid stress, a yield of 0.42% was found for gamma-linolenic acid C18:3 ɷ6c (6, 9, 12). When JNU532 was subjected to alkaline stress conditions, the production of C20:1 ɷ9c and C20:1 ɷ7c was 0.24% and 0.39% (Table 2; Fig. 4).
The adhesion capacity of bacterial strains is influenced by several variables, including the surrounding environment, the physical and chemical characteristics of the bacterial surface, and interactions with other organisms. The adhesion capacity of bacterial strains is influenced by several variables, including the surrounding environment, the physical and chemical characteristics of the bacterial surface, and interactions with other organisms. Studies have indicated that the adhesion of LAB to colon cancer cells may be related to the fatty acid composition of their membranes (Bautista-Gallego et al., 2013). Under mildly acidic stress, the proportion of cyclopropane fatty acids (C19:0 cyclo ɷ8c) in the membrane increases significantly. Specifically, the fluidity and acid resistance of the cell membrane significantly influence the bacteria’s adhesion ability. Increased membrane fluidity makes receptors on the cell surface more accessible for interaction with ligands on the host cell surface. Enhanced acid resistance allows the bacteria to remain stable in acidic environments, improving their survival and adhesion capabilities. Therefore, cyclopropane fatty acids promote LAB adhesion to colon cancer cells by altering the physical and chemical properties of the membrane. Additionally, environmental stress may affect the fatty acid composition of LAB cells, thereby influencing their surface characteristics. In brief, this study evaluated the response of JUN532 under challenging conditions, including mild heat stress (42°C), slight osmotic pressure (3% NaCl), alkalinity (pH 8), and acidity (pH 4), compared to the control group (37°C). The results showed that acidic (pH 4) and alkaline (pH 8) conditions had no effect on the survival rate of JUN532, while the adhesion capability of JUN532 was strongest under mildly acidic stress. By analyzing the fatty acid composition, this study provides direction for future research on the metabolic mechanisms of JUN532, and verifies its basic physiological roles in the host’s gut, such as stress resistance and adhesion capability. Moreover, it establishes a theoretical foundation for the application of JUN532 in the dairy and food industries. The adhesion capacity of bacterial strains is influenced by several variables, including the surrounding environment, the physical and chemical characteristics of the bacterial surface, and interactions with other organisms.
Studies have indicated that the adhesion of LAB to colon cancer cells may be influenced by the fatty acid composition of their membranes (Bautista-Gallego et al., 2013). Under mildly acidic stress, the proportion of cyclopropane fatty acids in the membrane increases significantly. This alteration enhances membrane fluidity and acid resistance, which are critical factors affecting bacterial adhesion. Increased membrane fluidity facilitates the accessibility of cell surface receptors for interaction with ligands on the host cell surface. Enhanced acid resistance allows the bacteria to maintain stability in acidic environments, thereby improving their survival and adhesion capabilities. Consequently, cyclopropane fatty acids promote LAB adhesion to colon cancer cells by modifying the physical and chemical properties of the membrane.
Furthermore, environmental stress may alter the fatty acid composition of LAB cells, thereby influencing their surface characteristics. This study evaluated the response of JUN532 under challenging conditions, including mild heat stress (42°C), slight osmotic pressure (3% NaCl), alkalinity (pH 8), and acidity (pH 4), compared to a control group (37°C). The results demonstrated that acidic (pH 4) and alkaline (pH 8) conditions had no effect on the survival rate of JUN532. However, its adhesion capability was strongest under mildly acidic stress, indicating optimal adaptation to such conditions. By analyzing the fatty acid composition, this study provides insights into the metabolic mechanisms of JUN532. It also verifies the strain’s responses to various environmental stresses encountered in the host gut, such as changes in cell surface characteristics and adhesion capability. This research establishes a theoretical foundation for the application of JUN532 in the dairy and food industries.
Increased membrane fluidity makes receptors on the cell surface more accessible for interaction with ligands on the host cell surface. Enhanced acid resistance allows the bacteria to remain stable in acidic environments, improving their survival and adhesion capabilities. Therefore, cyclopropane fatty acids promote LAB adhesion to colon cancer cells by altering the physical and chemical properties of the membrane. Additionally, environmental stress may affect the fatty acid composition of LAB cells, thereby influencing their surface characteristics. In brief, this study evaluated the response of JUN532 under challenging conditions, including mild heat stress (42°C), slight osmotic pressure (3% NaCl), alkalinity (pH 8), and acidity (pH 4), compared to the control group (37°C). The results showed that acidic (pH 4) and alkaline (pH 8) conditions had no effect on the survival rate of JUN532, while its adhesion capability was strongest under mildly acidic stress, indicating the best adaptation in mildly acidic stress.
Conclusion
By analyzing the fatty acid composition, this study provides direction for future research on the metabolic mechanisms of JUN532. Additionally, it verifies the strain’s responses to various environmental stresses it may face in the host gut, such as changes in cell surface characteristics and adhesion capability. This research establishes a theoretical foundation for the application of JUN532 in the dairy and food industries.