Introduction
Global human consumption of edible insects has been widespread in the past, and remains so currently, especially in Asia and on the African continent (Feng et al., 2018). However, negative perceptions persist about the intake of edible insects, even though they have a comparable nutritional value in human diets to that of meat and poultry in Western countries (Park et al., 2017; Van Huis, 2013). This prejudice has led some researchers to consider how to avoid negative perceptions about consuming edible insects through modifying their appearance (Yi et al., 2013). Because Tenebrio molitor (TM; yellow mealworm) is an edible insect that is widely used as a food resource in the Western world (van Broekhoven et al., 2015), many studies have considered the technical functionality of mealworm species, such as emulsifying capacity, emulsion stability, foaming ability, foaming capacity, and processing suitability (Andersen, 2002; Caparros Megido et al., 2018; Kim et al., 2016; Yi et al., 2013; Yi et al., 2016; Zhao et al., 2016). However, protein functionality of other species of edible insect which was commonly used in Asia was not conducted. In Asian countries, Allomyrina dichotoma (AD) is commonly used in traditional medicine; the larval extract can be used as a source of antineoplastic agents and antioxidants, and has anticytotoxic properties (Suh et al., 2010). Protaetia brevitarsis seulensis (PB) is widely distributed in Asia, and is also used in traditional medicine (Lee et al., 2017). However, there is little information about the technical functional properties of AD and PB. In this study, two species of edible insect was used to compare extracted protein technical functionality with TM.
Removing the indigestible fraction from edible insects is a major consideration in their use as a source of protein (Choi et al., 2017; Mishyna et al., 2019). Researchers have approached extracting protein by the application of protein solubility using various processes, such as alkaline, enzymatic hydrolysis, or salinity methods even there were spent heavy cost and time to extract protein from edible insect (Nongonierma and FitzGerald, 2017). To date, studies have focused on determining extracted protein solubility and functional properties using methods based on pH or temperature control (Zhao et al., 2016). According to Choi et al. (2011), muscle protein is an important component of meat products because it controls gel formation properties and comprises over 50% of the salt-soluble myofibrillar proteins. Although many researchers tried to replace meat protein to edible insect protein, there is little information about the functional properties of salt-soluble proteins obtained from edible insects. Therefore, functional properties of extracted proteins from edible insect by saline solution have to be conducted.
The purpose of this study was therefore to evaluate the functional properties of extracted protein from three different edible insect species, to enhance their physicochemical properties as a food source according to protein solubility.
Materials and Methods
Three different species of freeze-dried edible insect were obtained from a commercial market (Farm bang, Jeongeup, Korea) at the larval stage, as follows: TM (moisture: 4.83±0.05; protein: 58.44±2.89; fat: 25.33±0.65; ash: 4.29±0.22), AD (moisture: 7.18±0.21; protein: 49.68±0.54; fat: 24.54±0.11; ash: 2.20±0.30), and PB (moisture: 4.00±0.05; protein: 51.08±0.40; fat: 21.18±0.18; ash: 5.24±0.15). For the protein extraction, 200 g of ground insect matter was dispersed in n-hexane (Daejung, Busan, Korea) at 1:5 (w/v). After stirring at 20°C for 1 h, fat dissolved in the hexane was removed; the procedure was then repeated until clear hexane was achieved. Hexane residue in the defatted insect powder (DIP) was then volatilized at 20°C overnight in a fume hood. The DIP was stored at −20°C prior to extraction.
Protein extraction from the DIP was conducted using 0.02% (w/v) ascorbic acid for water-soluble protein, and 0.58 M saline solution (0.49 M NaCl, 17.8 mM Na5P3O10, and 1 mM NaN3; pH 8.3, 2°C) for salt-soluble protein. DIP and each of the buffers were homogenized at 1:2 (w/v) at 10,000 rpm, and filtered using medical gauze. The filtrate was centrifuged at 15,000 g for 30 min at 2°C, and the resulting supernatant was considered to be an extracted protein solution without pH adjustment (Choi et al., 2011; Yi et al., 2016). Thus, water-soluble (W) and salt-soluble (S) protein solutions were obtained for TM, AD, and PB, which we termed WTM, STM, WAD, SAD, WPB, and SPB.
The compositional properties of defatted edible insects were determined using the standard reference methods of the AOAC (2000). Moisture content (AOAC method 950.46B) was determined by weight loss after 12 h of drying at 105°C in a drying oven (SW-90D; Sang Woo Scientific Co., Bucheon, Korea). Fat content (AOAC method 960.69) was determined by Soxhlet extraction using a solvent extraction system (Soxtec® Avanti 2050 Auto System; Foss Tecator AB, Höganäs, Sweden). Protein content (AOAC method 981.10) was determined by the Kjeldahl method (Kjeltec® 2300Analyzer Unit; Foss Tecator AB, Höganäs, Sweden). Ash was determined according to AOAC method 920.153.
Edible insect protein solubility was determined by the biuret method. After extracting protein with the buffers (0.02% (w/v) ascorbic acid and 0.58 M saline solution), the protein concentration of each solution was determined (Kim et al., 2017).
After mixing 0.5 mL of 1% extracted protein solution with 10 mL of 6 M HCl in a 20 mL ampoule, sealed samples were placed in a drying oven (SW-90D; Sang Woo Scientific Co., Bucheon, Korea) at 105°C for 24 h to hydrolyze under nitrogen. After evaporation to dryness at 40°C under vacuum conditions, the hydrolysates were dissolved in 3–5 mL of 0.02 M HCl. The amino acid content was determined using an amino acid analyzer (L-8800; Hitachi, Tokyo, Japan) using an ion exchange resin column (4.6 mm i.d.×60 mm) after filtering the hydrolysate using a 0.20 μm membrane filter (Merck KGaA, Darmstadt, Germany). The standard amino acids were obtained from Sigma-Aldrich Co. (St. Louis, MO, USA).
The protein concentration of samples was calculated using Bradford reagent (Sigma-Aldrich Chemical Co., St. Louis, MO, USA). Sodium dodecyl sulfate–polyacrylamide gel electrophoresis (SDS-PAGE) was performed as described previously (Yi et al., 2013). Briefly, the protein samples (20 μg) and sample buffer (Bio-Rad Laboratories Inc., CA, USA) were mixed together at a ratio of 1:1. Then, the mixtures were heated at 100°C for 5 min, and separated using 10% SDS-PAGE. Next, the gel was stained with Coomassie Brilliant Blue R250 (B7920; Sigma-Aldrich, St. Louis, MO, USA), and the stained protein bands were identified by molecular weight (kDa) using a regular range protein marker (PM2510; SMOBIO Technology Inc., Hsinchu City, Taiwan) (Kim et al., 2018).
The pH value of each dissolved protein solution was measured using a Model 340 pH meter (Mettler Toledo GmbH, Schwerzenbach, Switzerland).
The instrumental color of protein solution extracted from the three edible insect species was determined using a colorimeter (CR-410 Chroma Meter; Konika Minolta, Osaka, Japan). A white plate was used for calibration. Plate conditions were as follows: CIE L* (lightness), 97.83; CIE a* (redness), –0.43; CIE b* (yellowness), 1.98; and observer orientation, 2°. After pouring 1% extracted protein solution into the CR-A40 colorimeter to a height of 2 cm, instrumental color (L*, a*, and b*) was detected.
After the protein concentration of the solution adjusted to 1% (w/v), 10 mL of each sample was transferred to a 50 mL conical tube. Each solution was homogenized at 12,000 rpm for 2 min to produce foam. Foam capacity was calculated as the percentage difference between the initial and foaming solution volumes. After completion of homogenization, the foaming solution volume was recorded at 2, 5, 10, 20, 30, and 60 min (Mishyna et al., 2019).
In order to determine the emulsifying capacity, 10 mL of 1% (w/v) protein solution and 1 mL pure olive oil was homogenized at 18,000 rpm for 2 min. After holding for 10 min at 20°C, the volume of the emulsified oil layer was measured. The difference between the volume of solution before and after homogenization was calculated as a percentage. 50 μL of emulsion and 10 mL of 0.3% (w/v) SDS solution were mixed to determine the emulsion stability. After the solution was inverted a few times, a holding time at 10, 20, 30, 60, 90, and 120 min was applied. A spectrophotometer was used to measure the absorption of each solution at 500 nm, and the difference before and after the holding time was calculated as a percentage to determine emulsion stability (Pearce and Kinsella, 1978).
Significant differences between treatments were calculated using one way analysis of variance with Duncan’s multiple range test (p<0.05), using SPSS statistical software (Version 20.0; IBM Corp., Armonk, NY, USA). A t-test (p<0.05) was performed to compare protein solubility among extracted protein from different solutions and the same species. Regardless of the edible insect species or solution salt concentration, each treatment was considered as an independent variable when comparing amino acid composition, protein quality, pH, color, foaming capacity, and emulsifying capacity.
Results and Discussion
The proximate composition of the defatted edible insect matter is shown in Table 1. Proximate compositions were significantly different. The moisture content of TM was the lowest (p<0.05), and that of AD and PB was the highest (p<0.05). Protein content increased and fat content decreased notably after the defatting processing. TM had the highest protein content, followed by AD (p<0.05); PB had the lowest protein content. TM had the highest and AD the lowest fat content (p<0.05). The ash content of the three species showed that PB had the highest and AD had the lowest (p<0.05). Finally, comparisons before and after defatting processing revealed wide changes in protein and fat content; increased protein content could be helpful when extracting protein (Yi et al., 2013). The effect of the different buffer solutions on protein solubility is shown in Table 1. As referred to in the discussion of protein solubility in the Materials and Methods, protein concentrations were calculated after extracting protein from the defatted edible insect powder, using the biuret method. Protein solubility can be affected by pH, salinity, and temperature because of changes in the net charge of the protein (Mishyna et al., 2019). Furthermore, different protein concentrations can significantly affect the rheological properties of protein solutions. Therefore, protein solubility might be a major consideration in increasing the functional properties of proteins. Only AD showed a significant difference between solutions (p<0.05), whereas TM and PB showed similar protein concentrations between extractions with different solutions (p>0.05). When extracted using 0.02% ascorbic acid solution, the protein concentration of AD was the highest, followed by PB, with TM having the lowest concentration (p<0.05). Although the protein solubility of AD decreased when extracted using 0.58 M saline solution, compared with the protein concentration achieved by 0.02% ascorbic acid solution, its protein concentration was the highest (p<0.05). The protein concentration of PB was not significantly different from AD (p>0.05), and that of TM was the lowest (p<0.05).
The amino acid composition profile and protein quality of the extracted edible insect proteins are shown in Table 2. Many types of essential amino acid (EAA) derived from 1% extracted edible insect protein solution are unable to provide the EAA levels required for humans, except histidine (FAO/WHO/UNU, 1985). Total EAA was the highest in SPB (151.62±3.86 mg/g) and the lowest in STM (68.52±1.30 mg/g); the total EAA value was close to that for STM in SAD (71.99±1.99 mg/g) and WTM (69.07±1.79 mg/g) (p<0.05). If these protein solutions were concentrated and used in powder form, the EAA of the extracted protein powder would satisfy human consumption requirements. According to Ghosh et al. (2017), TM dried matter had the highest value for total EAA, and PB the lowest value. In our study, PB had a higher value for total EAA than other species (p<0.05). This result might indicate that extracted protein of PB had suitable position than that of other insect by buffer regardless of salt concentration and extracted protein of TM had an unfit position. Furthermore, SPB also had the highest value for total amino acids (TAA) (p<0.05), followed by WPB. According to the essential amino acid index, SPB had the highest value for protein quality, followed by WPB and TM, and the lowest value regardless of solubility (p<0.05). Therefore, the extracted water- and salt-soluble edible insect proteins were shown to have a better protein quality in PB than in TM and AD.
Soluble proteins from the three different edible insect species were divided by SDS-PAGE using 10% separation gel under non-reducing conditions, and classified into six groups according to species and solubility (Fig. 1). Protein function was determined by protein characteristics, such as distribution of molecular weight, amino acid composition, and amino acid sequence (Nalinanon et al., 2011). All protein bands of the six groups were shown, from 10 to 180 kDa; these molecular weights showed a greater difference among edible insect species than did protein solubility. Protein bands of the TM groups were found at around 10 to 15 kDa, and less than 25 kDa. According to Yi et al. (2013), water-soluble protein bands of TM were observed to be abundant at below 14 kDa; these bands can be derived from anti-freeze proteins (range, 8.5 to 13 kDa) and hemolymph proteins (less than 12 kDa). AD groups had protein bands that were focused at around 25 kDa. These bands might originate from insect cuticles, the protein bands of which have been observed in the range of 14 to 32 kDa (Andersen et al., 1995; Dong et al., 2016); fainter bands were also observed at 35, 45, 75, and 180 kDa. However, PB groups showed a noticeable difference in molecular weight according to solubility. The WPB protein band was abundant in the range of 10 to 25 kDa and at 35 kDa. In contrast, the SPB protein band was abundant from 35 to 180 kDa. The muscle proteins, myosin heavy chain (205 kDa), actin (45 kDa), and tropomyosin (34 kDa), can be dissolved in saline solution (Choi et al., 2011). Compared with other species, PB muscle proteins dissolved abundantly, and no protein band was observed at at 25 kDa. Therefore, PB could be a good technical functional source when dissolved in saline solution.
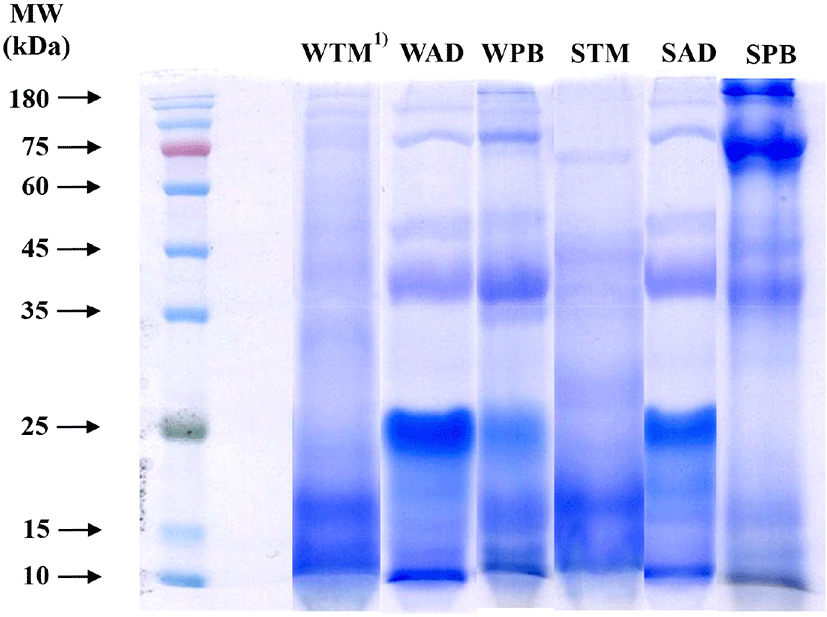
The pH and instrumental color of extracted edible insect proteins are shown in Table 3. When comparing the three species, PB had the highest and TM the lowest pH values (p<0.05). In general, water-soluble protein extracted solution had a lower pH value than salt-soluble protein extracted solution (p<0.05), because saline solution has a higher pH value (pH 8.0) than 0.02% ascorbic acid solution (pH 3.0). Protein solubility can be affected by pH and salt concentration, and the edible insect protein extraction rate is higher under alkaline conditions (Choi et al., 2011; Mishyna et al., 2019). Therefore, a weakly alkaline saline solution might be useful when extracting protein from edible insects. Although the protein concentration of all extractions was adjusted to 1%, they showed significant differences in L*, a*, and b*. According to Mishyna et al. (2019), different melanin contents by species of edible insect determined color difference among species. In this study, color of edible insects also was different by species. L* value of PB was lower than for that of TM and AD (p<0.05), and salt-soluble protein extracted solution had a lower L* value than water-soluble protein extracted solution, regardless of insect species (p<0.05). When comparing a* among species, PB had the highest and AD the lowest value (p<0.05); the S group had a higher value than the W group (p<0.05). The W group had higher b* value than the S group, and AD had a higher b* value than the other species (p<0.05). According to Mishyna et al. (2019), alkaline conditions can cause L* and b* to decrease and a* to increase, because phenolic compounds in insect cuticles can undergo oxidation, and occur protein–phenolic interaction (Atkinson et al., 1973). A similar phenomenon occured when canola and rapeseed proteins are extracted in alkaline conditions (Nadathur et al., 2016).
Foaming capacity and foam stability of extracted protein solution from different species of edible insect are shown in Fig. 2. Foaming capacity differed among species and solubility categories (Fig. 2A). WPB, SPB, and SAD produced the highest foaming capacities, followed by WAD and STM (p<0.05). WTM showed the lowest foaming capacity (p<0.05). The difference of foaming capacity can be explained by protein structure, concentration, and ionic strength (Yi et al., 2013). Furthermore, protein functionality of extracted protein was higher when pH of extracted protein was higher (Mishyna et al., 2019). Hihger pH value of PB might have positive effect on foaming capacity and tedency of foaming capacity was similar with tendency of pH value. As mentioned eariler, molecular weight distribution of PB also was fit for enhancing functionality. Therefore, foaming capaicty of PB was the highest with compositive reason such as pH or molecular weight distribution. Foam stability showed extreme differences in treatment (Fig. 2B). SPB was the most stable, followed by STM and WPB. SPB maintained an emulsion stability of over 50% for more than 50 min, and STM and WPB maintained a similar level for 25 min. SAD, WAD, and WTM showed a rapid callapse in stability, falling below 50% within 5 min. There were three main mechanisms related to foam collapse: disproportionation and coalescence of bubbles, and water drainage to the liquid layer. Foam stability can be increased by hydrophobic amino acid content and residue location, surface hydrophobicity, thiol groups, cations and anions, carbohydrates, and lipids (Panpipat and Chaijan, 2017; Zielinska et al., 2018). According to protein distribution (Fig. 1), AD group had band at 25 kDa to be expected chitin and this component interrupts foam stability (Yi et al., 2013).
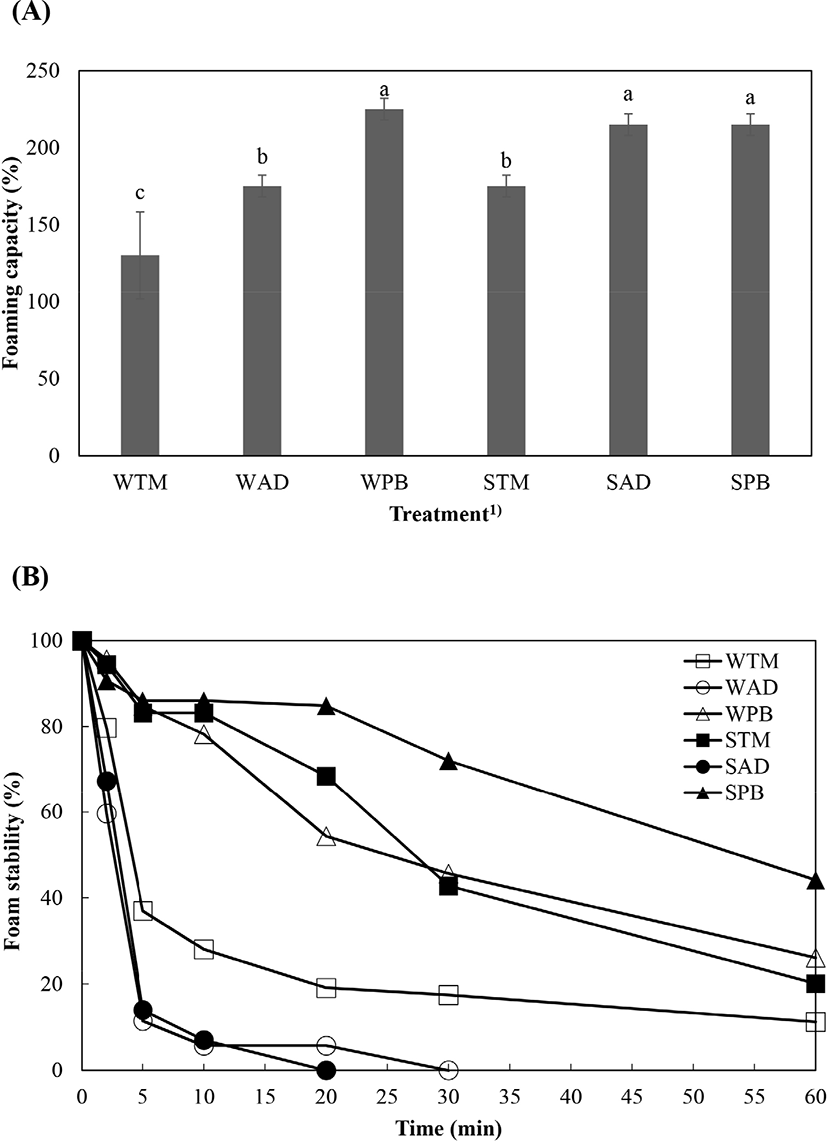
Emulsifying capacity and emulsion stability of extracted protein solutions from different edible insect species are presented in Fig. 3. SPB showed the highest emulsifying capacity, followed by WPB (p<0.05). WTM and AD groups had the lowest emulsifying capacity values (p<0.05). In particular, SPB had an emulsifying capacity of 100%. Differences in emulsifying capacity might be due to protein concentration, improved solubility, and protein hydrophobicity (Mishyna et al., 2019). SPB had a higher component of total hydrophobic amino acid than other solution such as Gly, Ala, Val, Leu, Ile, Pro, Phe, Met, and Trp (Table 2); its emulsifying capacity was therefore the highest because high hydrophobicity can affect protein adsorption, and interfacial tension reduces with droplet break-up (O’Sullivan et al., 2016). However, SPB did not have the highest emulsion stability; the emulsion stability of WTM and SAD was higher than for SPB at all times. In this case, the lower molecular weight of WTM and SAD might influence their emulsion stability (Mishyna et al., 2019). In contrast, WAD showed a rapid collapse in emulsion stability when compared with other treatments. This phenomenon might be due to its abundant insoluble protein fraction (25 kDa) (Fig. 1).
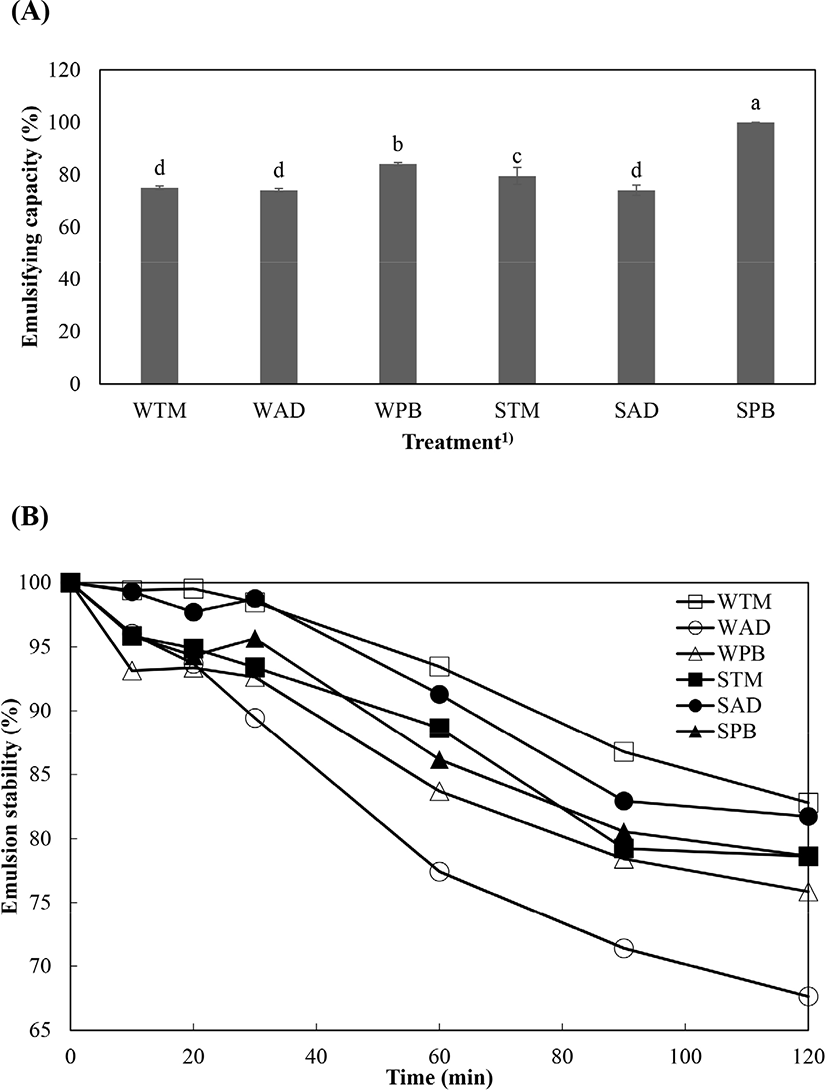
Conclusions
Our results showed that the technical functional properties of edible insects differed depending on insect species and type of buffer solution. AD had the highest protein solubility. However, its technical properties were not good when comparing similar protein concentrations. Most previous studies have focused on TM. However, our study showed that PB has good potential as a source of technical functional protein when comparing its amino acid composition, protein quality, foaming capacity, and emulsifying capacity with other species. Furthermore, its extracted protein properties were higher when extracted using 0.58 M saline solution. In conclusion, PB is promising as a good functional protein source when extracted using 0.58 M saline solution.