Introduction
In recent decades, global demand for goat meat has increased. This is partly because goat meat is considered a “healthy food,” as indicated by its nutritional benefits, high-quality protein, and low undesirable fat (Kim et al., 2019; Mazhangara et al., 2019). Furthermore, goat meat is not restricted by any religion (Abhijith et al., 2021). As a result, the worldwide goat meat production has increased from 5.1 million tons in 1970 to 6.39 million tons in 2021 (Gawat et al., 2023). In Korea, there is a high demand for goat meat and its whole carcass (e.g., skin, meat, and bone), which is used as a precious material for the production of folk medicine products (e.g., in the form of extracts and concentrates; Kim et al., 2019; Park et al., 2020). Due to insufficient production yield, a large amount of goat carcasses must be imported from foreign markets; in 2021, for instance, Korea imported goat meat with a value of 187 million USD, becoming the world’s 14th largest goat importer (OEC, 2024).
Korean native black goats (KNG) and crossbred goat (CG) breeds are the two main goat breeds farmed for commercial purposes. Historical documents show that KNG was domesticated in Korea over 2,000 years ago (Kim et al., 2014). KNG, which have a black coat, are characterized by a small body and low growth performance (Son, 1999). For centuries, the KNG has been the main goat breed used for meat and traditional medicinal purposes. However, as mentioned above, because of low growth performance and pressure from the increasing demand for KNG, foreign goat breeds have been introduced into the country decades ago. Among these, Boer is a specialized meat goat breed with high growth performance and muscling potential (Malan, 2000). Because of this advantage, Boer goats have usually been used as a terminal sire for crossbreeding with KNG to generate CGs with improved growth rates and meat quality (Choi et al., 2023). To date, CGs have been distributed and raised on more than 14,000 farms in South Korea (MAFRA, 2019).
Meat is an integral protein source in the human diet. Meat quality has a complex definition that includes all aspects from technological [e.g., cooking loss and water holding capacity (WHC)], texture (e.g., tenderness), and color to eating quality attributes, which are the main factors determining consumers’ meat purchasing decisions (Purslow, 2017). Providing consumers and producers with scientific information on the quality and nutritional value of goat meat is important for promoting the production, consumption, and trade of this meat type. Compared with cattle, pigs, sheep, and poultry, limited scientific information is available regarding the factors affecting the quality and nutritional composition of goat meat (Chen and Liu, 2020; Pereira and Vicente, 2013; Wood et al., 2008). To date, few studies have reported the effect of pre-slaughter factors on the quality of goat meat. Choi et al. (2023) found an increase in shear force and CIE a* value of the Korean black goat longissimus lumborum (LL) muscle with increasing slaughter age from 12 to 36 months. However, no effect of increasing slaughter age on total fat content, water-holding capacity, or cooking loss was reported by these authors. Regarding feed, Kim et al. (2022) found no differences in the effect of an alfalfa hay diet and alfalfa hay supplemented with a concentrate (at an 8:2 ratio) on total fat content, pH, and color of the KNG LL muscle. Recently, Farid et al. (2023) found that neither sex nor slaughter age affected the shear force and the CIE a* and CIE b* values of goat longissimus dorsi (LD) muscle. However, little is known about the effect of crossbreeding on the quality of goat meat. This study aimed to assess the effects of crossbreeding on the quality and nutritional composition of goat meat.
Materials and Methods
To determine the genetic origin of each breed, all DNA samples isolated from the LD muscle of each animal immediately after slaughter were sequenced using the single nucleotide polymorphism (SNP) technique. Based on the result (data not shown) of the SNP analysis, two breeds, purebred KNG and CG (1/4 KNG♀×3/4 Boer ♂) were confirmed and used for this study. For the KNG group, eight non-castrated males (average body weight 51.7±9.88 kg) and eight females (average body weight 37.14±5.16 kg) aged 32 months obtained from the Animal Genetic Research Center (National Institute of Animal Science, Hamyang, Korea) were used. For the CG group, eight non-castrated males (average body weight 55.21±6.82 kg) and eight females (average body weight 49.64±7.17 kg) aged 12 months obtained from a commercial goat farm (Gangjin, Korea) were used. The rearing conditions for both goat breeds were almost identical; the animals were grazed on pastures containing mixed plant species, provided ad libitum with hay, and supplemented with commercial feed (at approximately 1% body weight). The commercial feed was composed of crude protein (13%), crude fat (2%), crude fiber (20%), crude ash (10%), and calcium (0.7%). Goats were transported from the farm to a slaughterhouse at the National Institute of Animal Science (Korea) with a journey time of approximately 2 h. To minimize pre-slaughter stress, farm owners familiar with the goats transported the goats directly to the slaughterhouse using a specialized vehicle. After arrival, the animals were kept in a quiet lairage for approximately 2 h before slaughter. Slaughtering was performed during spring, and separate slaughter baths were used for each breed. After captive-bolt stunning, the animals were bled, the head was cut off, the carcass was steamed at 65°C for 5 min, dehaired with a mechanical machine, eviscerated to remove all internal organs, split, washed and chilled at 4°C for 24 h. Following the chilling, the LD muscles collected from both carcass sides were immediately used for analysis of meat quality and nutritional composition. Prior to analysis, each LD muscle was trimmed of visual connective tissues and prepared into subsamples depending on the analysis. To minimize variation in the results, the cutting method was the same for all samples in each analysis. Representative images of the goat muscle samples used in this study are shown in Fig. 1.
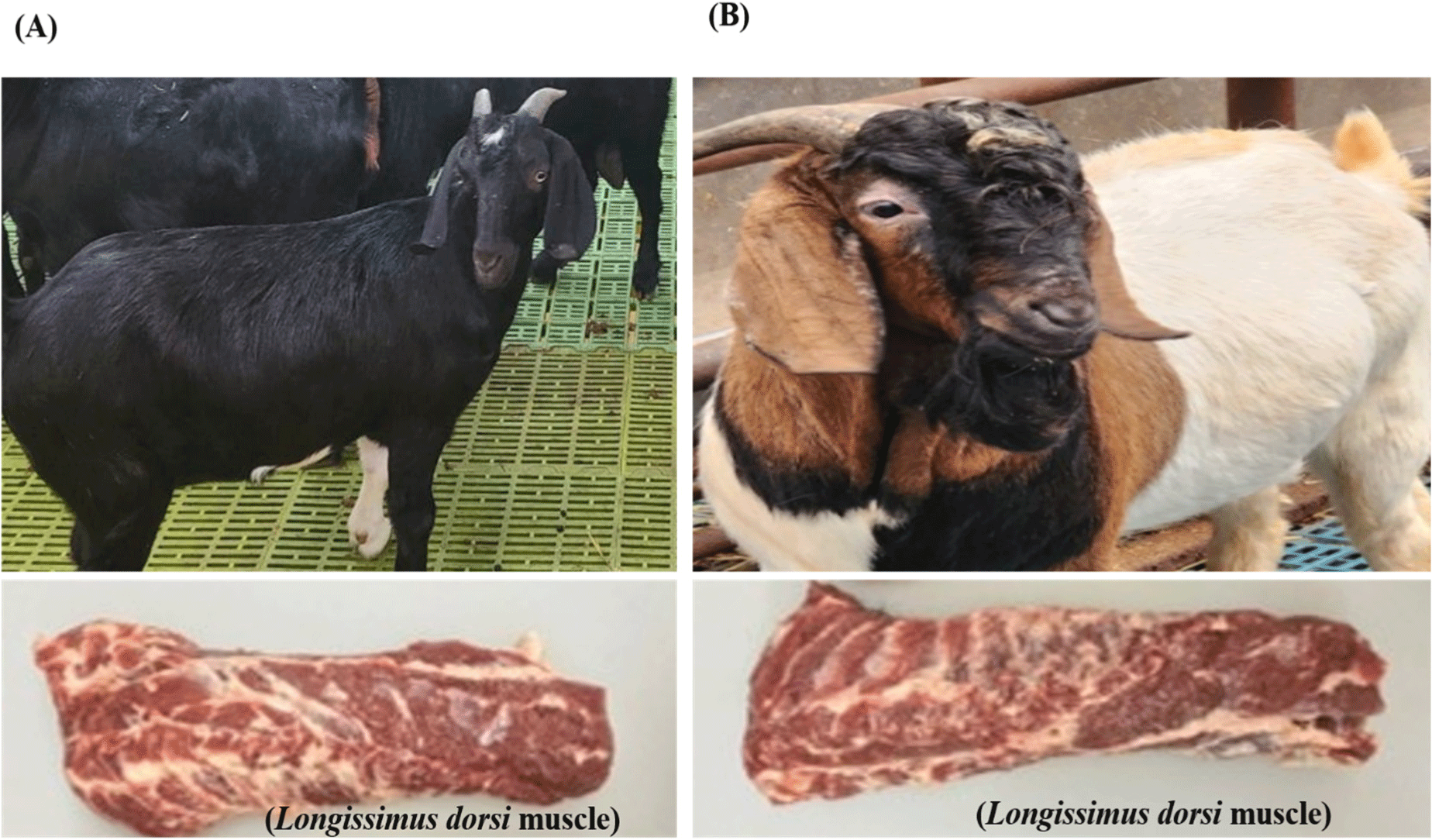
Moisture, fat, protein, ash and collagen contents in the samples were analyzed using a Food Scanner (model 78810, Foss Tecator, Hillerød, Denmark; Hoa et al., 2023a). The measured contents were expressed as percentages (%) of the total. Each sample was analyzed in duplicate.
For pH measurement, triplicate 3 g aliquots of each sample were weighed, placed in 50 mL conical tubes, and homogenized with 27 mL of distilled water for 30 s at 300×g. Ultimate pH was measured using a pH meter (Mettler-Toledo, Greifensee, Switzerland). Before use, the device was calibrated with standard pH solutions, according to the manufacturer’s instructions.
Color traits were measured across freshly cut surfaces of the meat samples using a color meter (CR-400 with a D65 illuminant and 2° observer, Konica Minolta, Osaka, Japan). Each sample was measured on three representative locations of sample 30 min after cutting at 4°C. Five color traits measured were CIE L*, CIE a*, CIE b*, chroma and hue angle (h°). The chroma and ho were calculated as (CIE a*2 + CIE b*2)0.5 and tan–1 (CIE b* / CIE a*), respectively (Seong et al., 2014).
To determine the cooking loss, each sample in the form of 2.5 cm thick steak (n=2 per sample) was cooked to a core temperature of 75°C using a pre-heated water bath (model: WSB-45, Daihan Scientific, Seoul, Korea). The core temperature was monitored using a thermo-recorder (TR-71wf, T&D, Matsumoto, Japan). Immediately after cooking, the samples were cooled in ice-cold water for 30 min. The samples were wiped with paper towels and weighed to calculate the cooking loss using the following Eq. (1):
To measure Warner-Bratzler shear force (WBSF), each cooked meat sample was manually cut into strips (1.0×1.0 cm: width×height, 3–4 strips/sample) following the muscle fiber direction. WBSF values were obtained by cutting the strips using a Texture Analyzer (Model 4465, Instron, High Wycombe, UK).
The fatty acid profiles of the goat meat samples (10 g each) were analyzed after extracting the lipid content using a mixture of solvents (Hoa et al., 2023b). The lipids were carefully collected from the upper layer after filtering the extract through a Whatman filter paper. The samples were concentrated with a rotary evaporator at 55°C and methylated with 1 mL of 0.5N NaOH and 1 mL tricosanoic acid. Finally, methylated fatty acids were analyzed using a gas chromatograph (GC) equipped with a flame ionization detector (FID; Varian Technologies, Palo Alto, CA, USA). A capillary column (30 m×0.25 mm×0.25 μm film thickness; Supelco, Bellefonte, PA, USA) was used for the separation of fatty acids. The GC/FID conditions were the same as described previously (Hoa et al., 2023c). A standard mixture of fatty acid methyl esters (Sigma-Aldrich, St. Louis, MO, USA) was used to identify and quantify fatty acids in the samples. The standard mixture was separated using the same column and conditions and the retention times were compared with those of the samples. Each fatty acid was expressed as a relative percentage (%) of total fatty acids. Each sample was analyzed in duplicate.
The protocol described by Kang et al. (2020) was used to analyze the free amino acids (FAA) content in goat meat samples. Briefly, duplicate 20 g aliquots of each sample were weighed, placed in conical tubes, and homogenized in 10 mL of trichloroacetic acid (5% w/v). After centrifugation at 12,000×g at 4°C for 15 min, the supernatant was carefully collected and filtered through a 0.2-μm filter membrane (Advantec, Irvine, CA, USA). Thereafter, FAAs were derived using AccQ-TagTM (Waters, Milford, MA, USA) according to the manufacturer’s instructions. Finally, the derived sample (0.2 mL) was pipetted into and FAAs were analyzed. The FAAs were separated on a reversed phase column (Waters), connected with a liquid chromatography system (model: UltimateTM WPS-3000RS). Mobile phases A: 10% Waters AccQ-tag Eluent A concentrate and B: 100% Waters AccQ-tag Eluent A concentrate at flow rate of 0.4 mL/min were used to separate the FAAs. The detection was monitored at 260 nm. A set of amino acid standard mixtures (Sigma-Aldrich) separated under the same conditions was used to identify and quantify FAAs in the samples. The FAA concentration (mg/100 g meat) was calculated for each sample.
Sample preparation for vitamin analysis was performed according to the AOAC method (AOAC, 2000; AOAC, 2019). Then, the samples were analyzed using a high-performance liquid chromatography-fluorescence detector (Agilent, Santa Clara, CA, USA). The separation was performed using a LaChromUltra C18 (2 mm i.d×50 mm, 2 μm particle size, Hitachi High-Tech, Tokyo, Japan). The column temperate and flow rate were set at 40°C and 0.2 mL/min, respectively. A mobile phase of acetonitrile: methanol (50:50, v/v) was used for the isocratic elution. Each vitamin standard was diluted to different concentrations and separated under the same conditions. A standard curve was drawn and used for quantification. The vitamin content was calculated and expressed as mg/100 g of meat. Each sample was analyzed in duplicate.
An aliquot (500 mg) of each sample was weighed and placed in a vessel containing 2 mL of hydrogen hydroxide solution and 7 mL of nitric acid. The samples were then pretreated using a Titan MPS Microwave sample preparation system (PerkinElmer, Waltham, MA, USA). The minerals were analyzed using Inductively Coupled Plasma-Atomic Emission Spectroscopy (ICP-AES, Avio 500, PerkinElmer), following the procedure described by Kang et al. (2020). The ICP-OES conditions were set as follows: power, 1,500 W; pump speed, 2.5 rpm; and nebulizer flow (0.7 L/min). A series of mineral standards (ICP multi-element standard, Sigma-Aldrich) was used for quantification, and the results were calculated and expressed as mg/kg of meat sample. Each sample was analyzed in duplicate.
SAS Enterprise (version 7.1, SAS Institute, Cary, NC, USA) was used to analyze the results. All data were analyzed using one-way analysis of variance (ANOVA), where the goat breed was considered as the fixed main effect, and the results of meat quality traits and nutritional composition were set as dependent variables. Duncan’s multiple range test was used to determine differences in the means between the two breeds, and significance was set at p<0.05. The Xlstat program (version 2023 5.2.1413.0, Addinsoft, New York, NY, USA) was used to explore the relationships between the variables (selected quality traits and nutritional composition) and goat breeds using principal component analysis (PCA).
Results and Discussion
The chemical composition of goat LD muscle by breed is shown in Table 1. The mean moisture, fat, protein, ash, and collagen contents of the two breeds ranged from 72.05% to 73.38%, 5.55% to 6.74%, 19.53% to 19.69%, 2.00% to 2.44%, and 1.83% to 1.87%, respectively. The only significant difference between the two breeds was the fat content. In particular, the CG had higher fat levels than the KNG (p<0.05). Similarly, Tshabalala et al. (2003) reported a higher fat content in Boer goat meat (10.45%) than in indigenous goat breeds (7.9%). Thus, the increase in fat content observed in the CG meat could be a result of crossbreeding with a goat breed with a high fat accumulation potential such as the Boer. When compared to fat level reported for KNG loin (5.21%–5.62%) by Kim et al. (2022), the KNG LD muscle in the present study had a similar level. From the point of view of its effect on eating quality, fat is well recognized as the most important determinant of eating quality along with juiciness and tenderness that influence meat purchasing decision by consumers (Chung et al., 2018; Gotoh et al., 2018). However, from the perspective of health, an increased intake of ruminant meat containing high fat may be associated with adverse health outcomes, such as increased total blood cholesterol and cardiovascular disorders (Vargas-Bello-Pérez and Larrain, 2017).
Meat quality traits, such as pH, cooking loss, and WBSF, of goat meat by breed are presented in Fig. 2. After slaughter, anaerobic glycolysis results in the accumulation of acids and a decline in pH, which affects other meat quality traits, such as color and WHC (Chauhan and England, 2018). We observed a significantly lower pH in the GC samples than in the KNG samples (6.10 vs. 6.28, p<0.05). This could be related to differences in the rates of postmortem glycolysis or glycogen stored in the muscle between the two breeds. According to our analysis, the CG samples had higher total glycogen content (60.15 μmol/g) than the KNG samples (41.33 μmol/g; data not shown). In contrast to our results, Ivanović et al. (2014) reported no effect of breed on the pH of goat LD muscle.
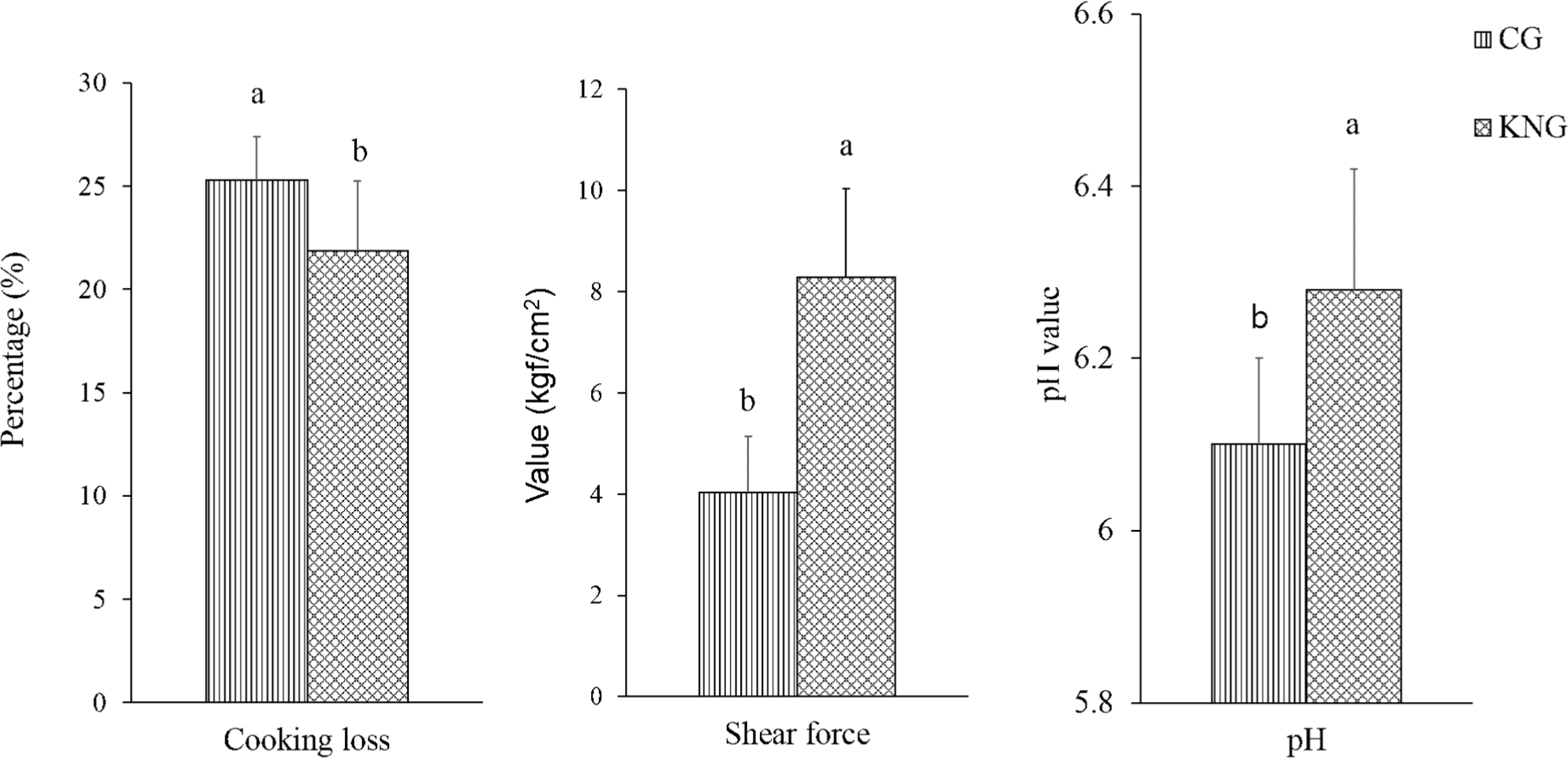
Cooking loss was significantly higher in the CG samples than in the KNG samples (25.3% vs. 21.85%, p<0.05). Gawat et al. (2023) reported a wide range of cooking loss (10%–27%) among goat breeds. The variation in cooking loss could be related to the differences in sampling, cooking conditions (time and temperature), and breeds used in these studies. Cooking loss affects the final meat yield after cooking. Cooking loss is an indicator of the WHC of fresh meat. Researchers have found that the ultimate pH largely affects the WHC of meat because low pH (acidic conditions) may cause protein denaturation, which reduces meat WHC (Huff-Lonergan and Lonergan, 2005). In the present study, the higher cooking loss in the CG samples may be related to their lower pH.
WBSF is the most common measure of meat tenderness; the higher the WBSF value, the tougher the meat. Our results show that the WBSF in the KNG samples was approximately two-fold higher compared to that of the CG samples (8.29 vs. 4.08 kgf/cm2, p<0.05), indicating that KNG meat is tougher than CG meat. To date, there have been few studies on the effects of crossbreeding on the quality of goat meat. However, differences in fat content (Table 1) may be the main factor responsible for the variation in WBSF values between goat breeds. Studies on beef have reported a negative correlation between fat content and WBSF (Hoa et al., 2022; Hoa et al., 2023a). However, older slaughter age could also be the reason for the higher WBSF value of KNG meat compared to that of CG meat. Choi et al. (2023) found an increase in WBSF values in goat LL muscles with increasing slaughter age. Boleman et al. (1997) classified meat tenderness based the WBSF, and values of 5.90–7.21 kgf/cm2 were considered tough meat. Similar to our results, high WBSF values ranging from 4.9 to 14.3 kgf/cm2 has been reported for goat meat under different cooking conditions and breeds (Gawat et al., 2023).
Color, which indicates the freshness and general status of meat, is the foremost factor affecting consumers’ selection of meat at the point of purchase (Purslow et al., 2020). Our results showed that breed had a significant effect on all color traits (Table 2). In particular, the CG samples exhibited higher CIE L*, CIE a*, CIE b*, chroma, and hue angle values than the KNG samples (p<0.05). This indicates that CG meat has a more attractive color (lighter and redder) than KNG meat. Similarly, to our results, numerous authors have also reported a significant effect of genotype on meat color in purebred and CG breeds (Yilmaz et al., 2009). The variation of fat content could be an appropriate explanation in the current study for the color difference between the two goat meat types. Indeed, studies on goat meat and the meat of other livestock species have shown that the higher the fat content, the lighter and redder the meat (Ivanović et al., 2020; Yim et al., 2015; Zhang et al., 2022). Furthermore, goat meat is characterized by a darker color because of its higher pH and lower marbling compared to other red meat types (pork and beef; Gawat et al., 2023). The lower CIE L* value of KNG samples can be attributed to its higher pH, as described above. In contrast to our results, Solaiman et al. (2012) found no differences in meat color (CIE L*, CIE a*, and CIE b* values) between the Boer and Kiko goat breeds.
The fatty acid content in meat is a very important component because it reflects not only the nutritional value but also significantly contributes to the flavor of cooked meat (Dinh et al., 2021). Our results showed that the KNG samples contained higher linolenic acid (C18:3n3), eicosenoic acid (C20:1n9), arachidonic acid (C20:4n6), total polyunsaturated fatty acids (PUFA), and n-3 PUFA than the CG samples (p<0.05; Table 3). The levels of total saturated fatty acids (SFA) and unsaturated fatty acids (UFA) ranged between the two breeds from 49.58% to 50.68% and 49.32% to 50.42%, respectively, without a statistical difference (p>0.05). In contrast, Choi et al. (2023) reported a lower SFA level in black goat LL muscle slaughtered at 12 and 36 months of age. Kim et al. (2022) reported a higher SFA content and lower UFA content in castrated KNG LD muscles slaughtered at approximately 58 months of age. The fatty acid composition (e.g., SFAs and UFAs) in meat is mainly affected by pre-slaughter factors such as diet and slaughter age (Wood et al., 2008). In the present study, we kept the factors (balanced sex and feeding regime) constant for both goat breeds, except for slaughter age. However, we assumed that slaughter age might not be the main factor affecting the fatty acid composition in goat meat. A number of studies on the meat of multi-gastric animal species, such as that conducted on Korean black goat meat, indicated that increasing the slaughter age from 3 to 36 months did not affect total PUFA content. Similarly, studies on cattle have shown that increasing the slaughter age from 1.9 years to 11.5 years (Cho et al., 2015), 26 months to 32 months (Dashdorj et al., 2012) or 60 months to 80 months (Utama et al., 2020) does not affect the total SFA and PUFA content in beef.
In the meat of multi-gastric animals (cattle and goats), UFAs are mainly formed in the de novo fatty acid biosynthesis pathway via SFA desaturation subsequent elongation (Malgwi et al., 2022). In the present study, the lower PUFA content in the CG meat may be related to the effect of crossbreeding, which slowed the conversion rate of SFAs to PUFAs during de novo fatty acid biosynthesis in this breed.
SFAs are among the major causative agents of increased total blood cholesterol and cardiovascular diseases (Vargas-Bello-Pérez and Larrain, 2017). However, researchers have demonstrated that not all SFAs have the same effect on cardiovascular diseases; the main SFAs are myristic acid (C14:0), palmitic acid (C16:0), and palmitoleic acid (C16:1n7; Grundy and Denke, 1990). The C14:0 and C16:0 contents of goat meat in the present study were lower than those of beef (Hoa et al., 2023b) and similar to those of pork (Hoa et al., 2021). In red meat, stearic acid (C18:0) is the second most abundant SFAs (Williams, 2007). Increased intake of C18:0 has been reported to reduce the risk of cardiovascular diseases (Thijssen and Mensink, 2005). Our results showed that the C18:0 content in both goat breeds was approximately 5%–6% higher than that in beef and pork (Hoa et al., 2021; Hoa et al., 2023c). Monounsaturated fatty acids (MUFAs), especially oleic acid (C18:1n9), play an important role in reducing the risk of cardiovascular diseases and the development of desirable flavors in meat during cooking (Smith, 2016). The total MUFAs (45%–47%) and oleic acid (43%–45%) in our study were similar to those reported for goat meat, but higher than the levels reported for grass-fed beef (40.09% oleic acid), pork (37%–41% oleic acid), and broiler chicken breast (32%–34% oleic acid) in previous studies (Al-Baadani et al., 2023; Choi et al., 2023; Hwang and Joo, 2017; Kim et al., 2019; Kim et al., 2022).
n-3 PUFAs are important constituents of cellular structures (mitochondria and cellular walls) and biological metabolism, and contribute to the protection of human health from cardiovascular diseases (Yashodhara et al., 2009). Although seafood is a well-known major source of PUFAs, meat is also an important source of dietary PUFAs (Vargas-Bello-Pérez and Larrain, 2017). When compared to the total n-3 PUFA content in beef (Hoa et al., 2022; Hoa et al., 2023a), both the CG and KNG meats had higher levels. However, the n-3 PUFA content in both goat breed meats was lower than that reported for pork and chicken (Al-Baadani et al., 2023; Hoa et al., 2023b). Consistent with our findings, Gawat et al. (2023) reported higher PUFA content in goat meat than in beef and sheep.
Essential amino acids (EAA) and non-essential amino acids (NAAs) are fundamental for protein synthesis. The nutritional value of meat is largely determined by the presence of EAAs. EAAs are those that the human body cannot synthesize via the de novo pathway and must be provided by dietary proteins (Church et al., 2020). Under the current experimental conditions, 17 FAAs, including eight EAAs and nine NAAs, were detected in the LD muscles of both breeds (Table 4). Of these, three EAAs (leucine, isoleucine, and threonine) were affected by the breed; KNG meat contained higher amounts of these EAAs (p<0.05). Regarding the NAAs, KNG meat also exhibited higher arginine, cysteine, serine, and tyrosine contents than CG meat (p<0.05). Interestingly, when compared to the concentrations of all EAAs and NAAs reported in beef, pork, and sheep in the literature (Ahmad et al., 2018; Hoa et al., 2023c; Park et al., 2008), the goat meat of both breeds contained a significantly higher amount (approximately 1.5 to 3 times higher depending on the amino acids).
In addition to serving as the building blocks of proteins, FAAs are important flavor-active components, as they significantly contribute to the taste (sweetness, umami, and saltiness) of cooked meat (Mateo et al., 1996). The FAAs that contribute to sweet taste are threonine, serine, glycine, and proline, whereas glutamic acid, lysine, methionine, and alanine contribute to the umami taste of cooked meat (Kato et al., 1989; Mateo et al., 1996). In addition, FAAs contribute to the development of aroma (e.g., meaty) via the Maillard reaction with reducing sugars during the cooking of meat (Khan et al., 2015). In muscle meat, FAAs content has been reported to be affected by pre-slaughter factors; animal breeds with a high growth rate usually have lower FAA availability because they are mostly used for protein synthesis (Koutsidis et al., 2008). Thus, the lower levels of some EAAs (e.g., leucine, isoleucine, and threonine) in CG meat may be attributed to the faster growth rate of this breed. Similar to our results, previous studies on beef have also found an effect of breed on the FAA content (Hoa et al., 2023a).
Vitamins are integrally important constituents involved in a variety of metabolic processes, growth, and maintenance of human health (Huskisson et al., 2007). Vitamin C and E content in the meat from the two breeds ranged from 0.36 mg/100 g to 0.41 mg/100 g and 0.11 mg/100 g to 0.19 mg/100 g, respectively (Table 5). Crossbreeding did not affect vitamin C, but did affect vitamin E, with a higher level in KNG meat (p<0.05). Researchers have reported that most vitamins are naturally present in plant-based diets, and some can be synthesized in the rumen by microbes (Rasikh, 2019). In this study, all goats were grazed on pastures containing mixed plant species; therefore, the difference in vitamin E content in meat may be related to different eating habits (e.g., purebred KNG may prefer a diet with a variety of plant species).
Cashman and Hayes (2017) reported vitamin E content of 0.1 mg/100 g in beef, pork and lamb, which is lower than that in our study. The vitamin E content of both goat breeds in our study was comparable to that of horse meat (Seong et al., 2019). Notably, vitamin C has not been found in other types of red meat (Ahmad et al., 2018); however, in our study, it was found in the meat of both goat breeds.
Minerals are generally classified into major minerals (e.g., Na, Ca, K, P, and Mg) and trace elements (e.g., Fe, Mn, Zn, and Cu) based on their daily intake (Gupta and Gupta, 2014). Major minerals are essential for sustaining basic functions, and their excessive or deficient intake may result in metabolic disorders. Our results showed that crossbreeding only affected the P content (higher level in KNG meat; p<0.05). In general, the major mineral contents of both goat breeds in the present study were similar to those reported for other meat types (beef, pork, and chicken; Ahmad et al., 2018; Pereira and Vicente, 2013).
Unlike major minerals, trace elements are required in considerably smaller amounts; however, they are vital for maintaining human health (Tapiero and Tew, 2003). We observed significantly higher Fe and Cu contents in KNG meat (p<0.05). The reason for the variation in mineral content could be related to the differences in muscle chemical composition (e.g., fat content; Table 1) between the two breeds. Compared to our results, Kim et al. (2019) reported lower levels of Fe (1.48 mg/100 g) and Ca (6.09 mg/100 g) in the rump cuts of male KNG. Interestingly, the concentrations of almost all trace minerals (Fe, Zn, and Cu) in goat meat of both breeds were much higher than those reported for beef, pork, chicken, lamb, and duck meat in previous studies (Ahmad et al., 2018; Pereira and Vicente, 2013). According to the recommended daily intakes of Fe (8 mg) and Mn (8–14 mg; Williams, 2007), consuming 100 g of goat meat provides over 30% and 100% of the Fe and Mn requirements, respectively. This implies that goat meat is a better source of trace minerals than other commonly consumed meat types.
The relationships between meat quality traits, nutritional composition, and goat breed were also explored using PCA. As shown in Fig. 3, KNG meat was on the negative PC1 axis; therefore, it was related to WBSF, fatty acids (C18:3n3, C24:4n6, PUFA, n6, and n3), amino acids (threonine, tyrosine, isoleucine, leucine, cysteine, and arginine), vitamin E, and minerals (P, Fe, and Cu). CG meat was on the positive PC1 axis; therefore, it was related to fat, C14:0, cooking loss, and color traits (CIE L*, CIE a*, CIE b*, chroma, and hue angle). The PCA results show variations in meat quality and nutritional composition between the KNG and CG meats, as presented in Tables 1, 2, 3, 4, and 5.
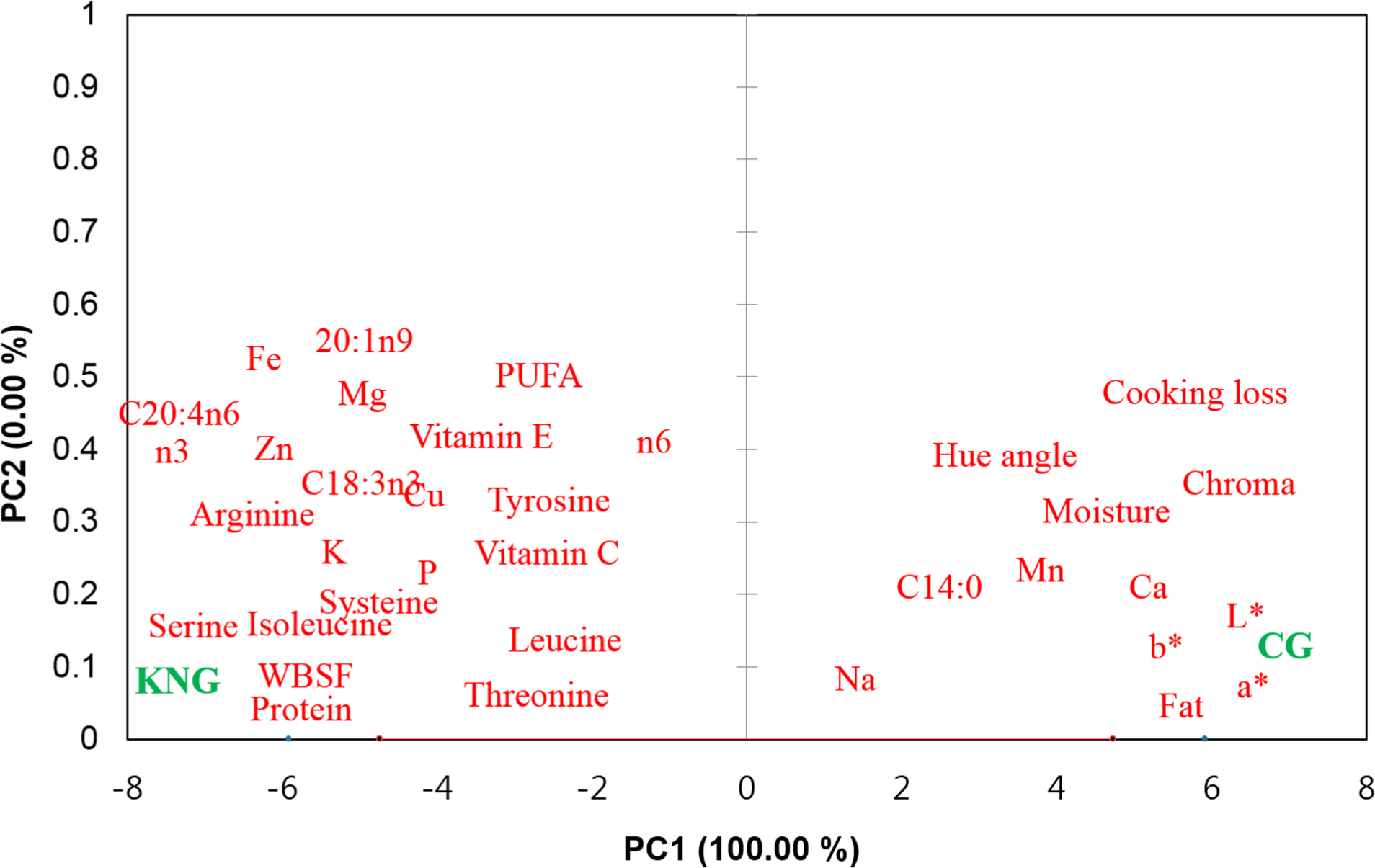
Conclusion
In this study, the effects of crossbreeding on the meat quality properties and nutritional composition of goat meat were investigated. In terms of meat quality, crossbreeding partly improved the color (lighter and redder in color) and tenderness. However, in terms of nutritional composition, crossbreeding reduced the total PUFA content, levels of some vitamins (e.g., vitamin E), and trace minerals (e.g., Fe and Cu) in meat. Crossbreeding also reduced the amount of some taste-active FAA in meat. Based on the results obtained from our investigations, crossbreeding improved quality traits; however, it also caused an increase in total fat content and a decrease in the nutritional value of this meat type.