Introduction
Proteins play a significant role in regulating numerous physiological processes including the endocrine, immune, circulatory, nervous, and digestive systems (Minkiewicz et al., 2008; Sobczak et al., 2023). Animal proteins are renowned for their high quality and ability to provide adequate and balanced amino acids (AAs); however, their functionality is limited by resources and processes (Wu, 2022). From a nutritional perspective, the incorporation of different-sourced proteins like plant-based proteins as well as the production of bioactive peptides by enzyme treatment can sufficiently meet human health requirements by providing an ample supply of essential AAs (EAAs) or enhancing AA absorption (Jeon et al., 2023; Kumar et al., 2022).
Efforts to replace livestock products are continuing because of the growing population and the lack of supply of animal proteins (Gerber et al., 2007). Despite the growing interest and research of plant protein-based alternatives, they have nutritional limitations that cannot completely replace animal proteins. Recently, yeast became preferable alternative protein sources in accordance with its well-established production process such as rapid growth and ease of harvest, high protein content, and low contamination risk (Lapeña et al., 2020; Øverland and Skrede, 2017). In addition, yeast proteins, can provide balanced AA composition with high solubility and water-holding capacity (Puligundla et al., 2020), which are important characteristics in playing an auxiliary role in livestock food.
The chemical or biological breakdown of proteins presents a promising approach for generating high-quality small and large peptides in the diets of livestock, poultry, and fish, providing both nutritional benefits and crucial physiological or regulatory functions (da Silva, 2018; Hou et al., 2017). Compared to chemical hydrolysis giving nonspecific breakdown into peptides and AAs, the enzymatic approach facilitates a highly precise and controlled cleavage of specific amide bonds (Oshimura and Sakamoto, 2017). In addition, it operates under mild reaction conditions, producing limited unwanted by-products (Czelej et al., 2022). For example, enzymatic protein hydrolysates play a significant role as supplements in livestock production. Numerous studies have investigated their impact on the growth performance and hematological parameters of beef cattle, as well as their effects on the health and performance of dairy cows, digestive function in cattle, and immune responses in calves (Gunun et al., 2022; Kim et al., 2011; Nocek et al., 2011; Salinas-Chavira et al., 2015; Stefenoni et al., 2020). Although substantial researches have been conducted on the use of various protein sources (Baker et al., 2022; Jach et al., 2022; Pang et al., 2022; Shurson, 2018), enzymatic hydrolysis of yeast protein remain relatively insufficient.
Based on positional specificity, proteolytic enzymes are categorized into two primary groups: endopeptidases and exopeptidases. Endopeptidases target internal bonds within polypeptides, whereas exopeptidases cleave near the C- or N-terminus (Gurumallesh et al., 2019). So far, enzymatic hydrolysis has been widely performed and their functional or structural alterations were reported (Chalamaiah et al., 2012; Dumitraşcu et al., 2023; Etemadian et al., 2021; Gajanan et al., 2016). Among them, several researches are employing various commercial proteolytic enzymes, including alcalase, neutrase, protamex, flavourzyme, pronase, and kojizyme of microbial origin; papain and bromelain sourced from plants; and pepsin, trypsin, chymotrypsin, and pancreatin derived from animals. However, it is still required to compare the hydrolysis effect using different groups of enzyme on structural and functional characteristics of yeast protein. Therefore, this study aimed to evaluate the effects of treatments using biological enzymes, either individually or in combination (endo- and exotypes), on the properties of yeast proteins during the production of enzymatic protein hydrolysates and provide fundamental data for exploring their potential application as an alternative animal protein.
Materials and Methods
Yeast protein was kindly supplied by Amored Fresh (Seoul, Korea). Proteolytic enzymes, including endopeptidases; alcalase 2.4 L FG (from Bacillus licheniformis) and neutrase 0.8 L (from Bacillus amyloliiensquefaciens) and exopeptidase; flavourzyme 1,000 L (from Aspergillus oryzae) from Daesang (Seoul, Korea) and prozyme 2000P from Bision Biochem (Seongnam, Korea) were supplied. Trichloroacetic acid (TCIchemical, Seoul, Korea) and bicinchonic acid (BCA) protein assay kit (Thermo Fisher Scientific, Rockford, IL, USA) were also used.
Two different types of commercial enzymes, namely, endo- (alcalase and neutrase) and exo- (flavourzyme and prozyme 2000P), were selected to hydrolyze the yeast protein. The details of these enzymes are presented in Table 1. Using 10% (w/v) suspension at 55°C using distilled water with the yeast protein, hydrolysis was conducted for 8 h at an enzyme/substrate ratio of 1 g enzyme/100 g protein (Suh et al., 2017; Xia et al., 2022). pH values were determined at 0, 1, 2, 4, 6, and 8 h. The samples were freeze-dried at −80°C and stored at room temperature before use for further studies.
Enzyme | Type | Optimal condition | Ref. |
---|---|---|---|
Alcalase 2.4 L FG | Endo | pH 6.5–8.5 55°C–70°C |
Noh et el. (2013) |
Neutrase 0.8 L | Endo | pH 6–9 30°C–60°C |
Zhang et al. (2022) |
Flavourzyme 1,000 L | Exo | pH 5–7.2 50°C–55°C |
Hau et al. (2022) |
Prozyme 2000P | Exo | pH 5–5.5 55°C–60°C |
Kim et al. (2022) |
The degree of hydrolysis (DH) of the protein hydrolysate was measured by following Park and Yoon (2018) with slight modification. In brief, one percent (w/v) of hydrolysate (with pH adjusted to 7) was followed by the addition of the same amount of 20% trichloroacetic acid solution. After conducting the reaction at room temperature for 30 min, centrifugation (Eppendorf 5910 R, Eppendorf, Hamburg, Germany) was performed at 2,634×g at 4°C for 20 min, and a supernatant was obtained. The absorbance of the supernatant was measured at a wavelength of 562 nm using an ultraviolet–visible spectrophotometer based on the BCA method (Smith et al., 1985) and the DH value was calculated (Ha et al., 2019).
Here, Wo and Wh represent the absorbance of yeast protein before and after hydrolysis, respectively.
An 1% (w/v) protein solution was incubated at a room temperature for 30 min at different pH from 2 to 12, which were adjusted using a 1 M HCl and 1 M NaOH solution, and centrifuged at 16,000×g for 25 min (Chen et al., 2018; Wang et al., 2021). The protein content in the supernatant was measured based on the BCA method. Protein solubility was expressed as a percentage value of soluble protein concentration to the total protein concentration of the sample.
Particle size distribution (PSD) was determined using a Mastersizer 3000 static laser light diffraction unit (Malvern Panalytical, Malvern, UK) across a size range of 0.01–3,500 μm by employing a red laser (633 nm) and blue light source (470 nm). Particle size is expressed as average passing values from the results presented in a volume-based PSD analysis using the Mastersizer 3000 software. The distribution width, often represented by the span, is calculated as (D90–D10)/D50, where D10, D50, and D90 denote the 10th, 50th, and 90th percentiles of the distribution, respectively (Istianah et al., 2024; Qin et al., 2023).
To analyze the chemical structure, the dried yeast protein was positioned on a Fourier-transform infrared spectroscopy (FTIR) plate (Nicolet iS5, Thermo Fisher Scientific, Waltham, MA, USA). Light absorption across wavelengths from 550 to 4,000 cm−1 was collected, and FTIR spectra were recorded using a spectrometer fitted with an iD7 ATR accessory with a ZnSe crystal (4,000–400 cm−1) at 25°C. The equipment was operated at a scan speed of 0.2 cm/s, and at 16 scans with a resolution of 4 cm−1. Background reference values were calculated using a standard log transformation of the sample and single spectra to remove the background signal. Their second-order derivative spectra were also obtained by using Origin Pro software (OriginLab, Northampton, MA, USA) after smoothing through the Savitzky–Golay algorithm employing nine data points from the analysis. The proportion of each secondary structural component is presented as a percentage, which is obtained by dividing the area of a single Amide I band component by the sum of the areas of all the amide band components.
The analysis of AAs within the yeast-protein extract was conducted using a Dionex Ultimate 3000 high-performance liquid chromatography system from Thermo Fisher Scientific, coupled with a 1260 Infinity fluorescence detector from Agilent Technologies (Waldbronn, Germany). The analysis method was based on the approach outlined by Min et al. (2023) and Yoon et al. (2019) with slight modifications. After the sample derivatization using o-phthalaldehyde (OPA) and 9-fluorenylmethoxycarbonyl (FMOC), 0.5 μL samples were injected into an Inno-C18 column (4.6×50 mm, 5 μm, Youngin Biochrom, Seongnam, Korea) at 40°C. Fluorescence detection was performed at excitation and emission wavelengths of 340 and 450 nm for OPA and 266 and 305 nm for FMOC, respectively. The primary and secondary AAs were identified using the OPA and FMOC derivatives, respectively. The mobile phases were as follows: 40 mM sodium phosphate (pH 7) as solvent A, 10:45:45 (v/v) mixture of distilled water, acetonitrile, and methanol as Solvent B. A gradient program was employed at a flow rate of 1.0 mL/min, starting with 5% Solvent B for 3 min, followed by a gradient from 5% to 55% Solvent B in 24 min and then from 55% to 90% Solvent B in 25 min. This concentration was maintained for 6 min before reverting from 90% to 5% Solvent B over 3.5 min, with a maintenance period of 0.5 min at 5% Solvent B.
Statistical analysis was performed using MINITAB version 21. All measured parameters were assessed using one-way analysis of variance, followed by Tukey’s post-hoc test to identify significant differences among the individual means. Statistical significance was determined at p<0.05.
Results and Discussion
The DH represents the percentage of cleaved peptide bonds in a protein hydrolysate and is a predominant parameter for distinguishing the structural variations among different hydrolysates (Yi et al., 2021). In this study, yeast protein gave over 80% hydrolysis yield after 8 h of enzyme treatment, regardless of enzyme types (Fig. 1A). The hydrolysis levels decreased in the order of neutrase, alcalase, prozyme 2000P, and flavourzyme, indicating endotype enzymes facilitate a higher hydrolysis efficiency in yeast proteins than exotype enzymes. The higher efficiency of endotype enzymes might be because of stronger product inhibition from exo products or lower activation energy for endo product (Furusawa et al., 2008). Considering the endotype enzyme treatments, the DH of neutrase (90.02%) was higher than that of alcalase (88.72%), which was consistent with the results of studies involving casein protein hydrolysate with the same enzyme employed in this study (Kim et al., 2021). After exotype enzyme treatments, the DH of prozyme 2000P (86.62%) was higher than that for flavourzyme (84.83%).
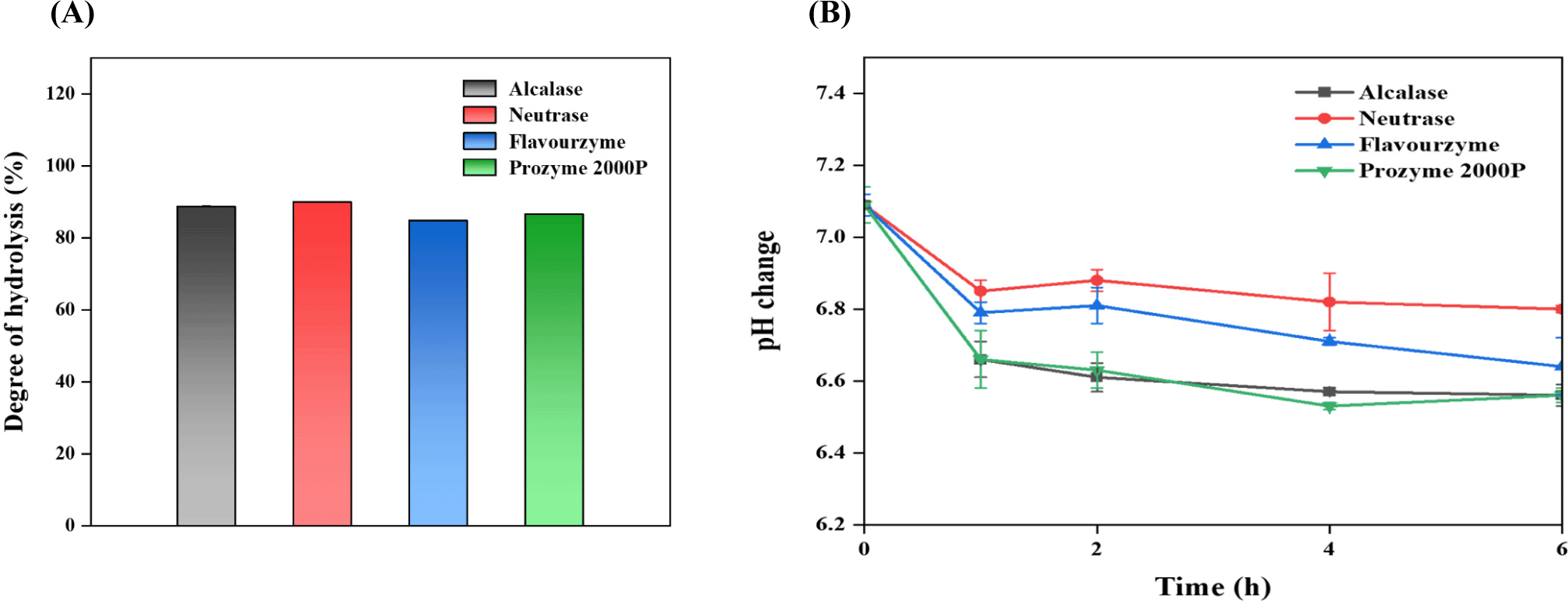
Meanwhile, regardless of enzyme types, the hydrolysis of yeast protein using endo and exo proteases was rapidly started right after the enzyme addition. It was indirectly proved by the changes in pH levels over time (Fig. 1B). For example, the initial pH of yeast protein (about 7.09) rapidly decreased within 1 h, and the pH variations became less significant over time, which is generally observed during protein hydrolysis, suggesting that rapid degradation within a short period may exert a positive industrial impact on peptide production (Suh et al., 2017). This decrease may be attributed by the protein degradation, leading to the accumulation of acidic AAs or the subsequently formed carboxyl groups (Gam et al., 2019; Ryu et al., 2015). Thus, the proteolysis of yeast protein might positively affect the final protein qualities, however be differently affected by the enzyme types.
Protein solubility, one of the typical criteria for measuring protein qualities, plays a crucial role in determining physicochemical properties, processing, nutritional profiles, etc. (Grossmann and McClements, 2023; Hellebois et al., 2021). Also, it largely affects formulation of products and their stabilities (Vihinen, 2020). Various intrinsic and extrinsic factors including molecular weight, specific AA composition, average charge, pH, and ionic strength collectively affect protein solubility (Diaz et al., 2010; Grossmann et al., 2019). In the present study, Fig. 2 illustrates the solubility of yeast proteins across diverse pH ranges. The yeast protein sample demonstrated the highest solubility at alkaline pH 12. Also, their solubility became notably high at acidic pH 2. Owing to the presence of a net negative or positive charge on a protein at high or low pH level (i.e. furthest above and below pI), a large amount of water might interact with the protein (Pelegrine and Gasparetto, 2005). Moreover, after enzyme treatment, the yeast protein exhibited a significant increase in protein solubility regardless of pH range, demonstrating an enhancement of more than three folds. The higher solubility of the protein hydrolysates than the initial proteins can be predominantly attributed to the liberation of polar functional gropus owing to the cleavage of peptide bonds. Especially, samples treated with neutrase exhibited the highest solubility among the enzyme-treated variants. Furthermore, as similarly to the hydrolysis results, samples treated with endotype enzyme including neutrase and alcalase demonstrated higher solubility than those processed with exotype enzymes. These findings are correlated with the results obtained from the hydrolysis of whey protein (Cui et al., 2021; Kim et al., 2022). In general, the protein solubilities are affected by both hydrophobic interactions among proteins and ionic interactions between protein and water (Cui et al., 2021; Xiong et al., 2023). Thus, hydrophilic structures that were previously concealed in the native structure of the aqueous solvent were revealed after enzyme treatment, which are increasing protein solubilities (Beaubier et al., 2021). The proteolysis of yeast protein might positively affect the final protein qualities in terms of enhancing solubility, however be differently affected by the enzyme types.
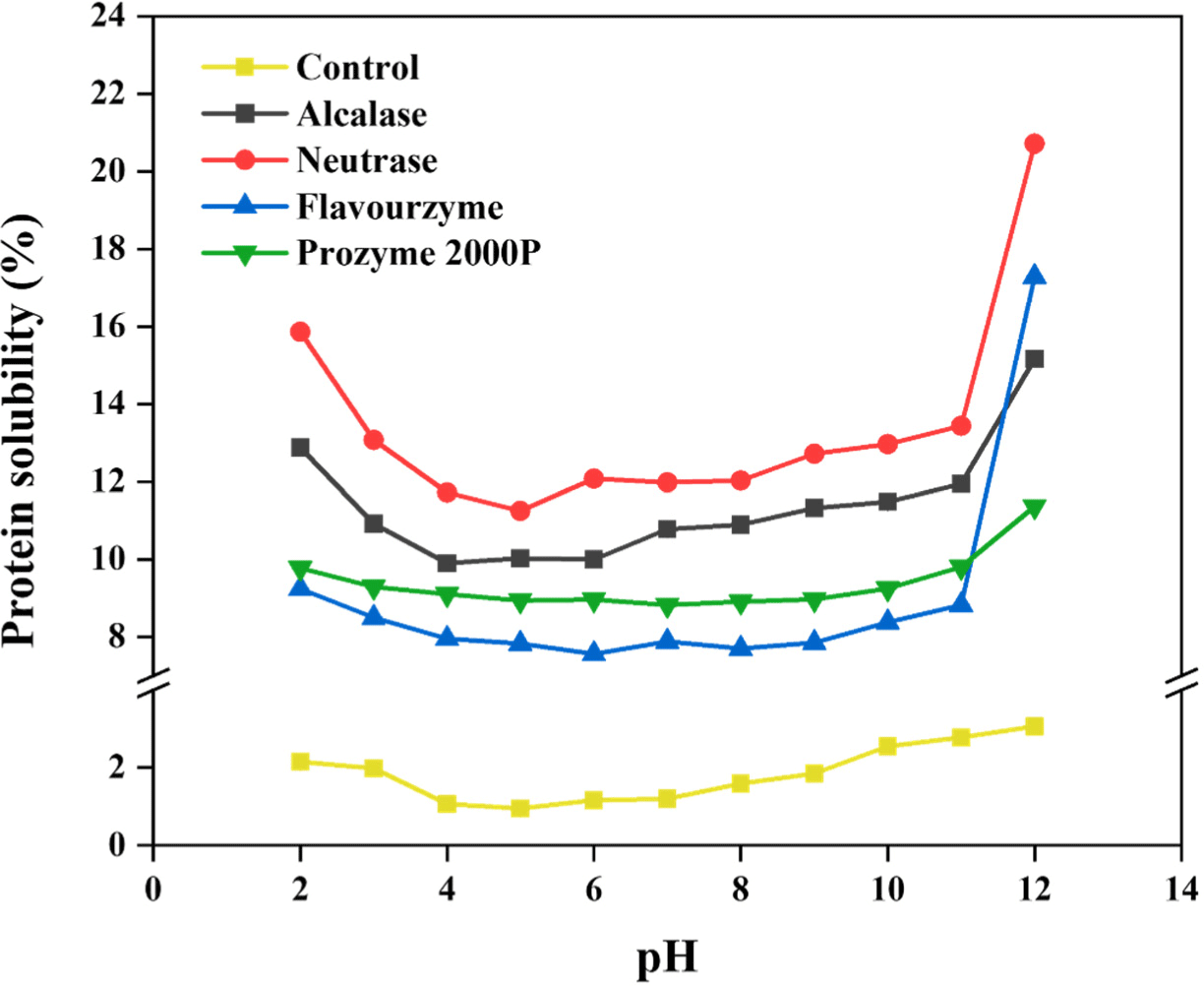
The particle size of food ingredients including protein samples is another important parameter indicating protein qualities. In general, a decrease in the particle size increases nutrient digestibilities by increasing available surface area (Blasel et al., 2006; Lyu et al., 2022). Table 2 illustrates the distribution of particle size of the protein hydrolysate after enzyme treatment. Yeast protein showed the average particle size (D50) of 12.80 μm with 3.71 μm D10 and 24.80 μm of D90. Enzyme treatment of yeast protein gave a decrease in the D50 value, which is generally observed from the hydrolysates of food proteins (Cui et al., 2021; Hao et al., 2022). The reduction in the protein sizes after enzymatic hydrolysis might be attributed to the disruption of protein structure, allowing smaller peptides to be more readily solubilized in the solution, thus correlating with an increase in peptide solubilities. The particle sizes were decreased in the order of prozyme 2000P, alcalase, neutrase, and flavourzyme, indicating the size reduction was not considerably affected by enzyme types. Alcalase in endo-type protease and prozyme 2000P in exo-type protease exhibited a lower particle size (9.96 μm; 9.44 μm, respectively), suggesting that the specific introduction of each enzyme or utilization of combinations of different enzymes were required, based on the diverse substrate compositions. Also, the limited particle size reduction (i.e. sizes in the μm range) could be attributed to the extent and duration of hydrolysis, which can lead to either further breakdown or aggregation of particles (Hao et al., 2022; Shen et al., 2020).
Although the particle size did not show consistency according to the employed enzyme types, the span values of endo- and exotype enzyme treatments indicating variations in D10 and D90 values were approximately 1.68 and 1.81, respectively. These smaller span values imply a higher degree of dimensional uniformity in the yeast protein after hydrolysis with a more consistent PSD (Jewiarz et al., 2020). Thus, with their lower span values, endotype enzymes treatment of yeast protein might contribute to a more uniform particle distribution, emphasizing their ability to promote particle uniformity.
The FTIR spectra (Fig. 3A) reveal the yeast protein contains characteristic peaks indicating Amide A, Amide I, Amide II, and Amide III. For example, a distinctive peak at 3,280 cm−1 corresponds to the N–H stretching vibration, which is a key absorption feature associated with Amide A (Haris, 2013; Zhou et al., 2016). The presence of Amide I and Amide II in yeast protein and its hydrolysate was confirmed by the appearance of peaks at 1,630 and 1,520 cm−1, respectively. The Amide I peak is attributed to the stretching vibration of C=O bonds and the Amide II peak is N–H and C–H stretching vibrations. In particular, Amide I exhibit the strongest transmission band and is highly sensitive to the secondary structure, reflecting diverse hydrogen-bonding environments associated with α-helix, β-sheet, turn, and unordered conformations (Prajapati et al., 2021). Furthermore, the bands at 2,930 cm−1 correspond to –CH2 groups (Gbassi et al., 2012).
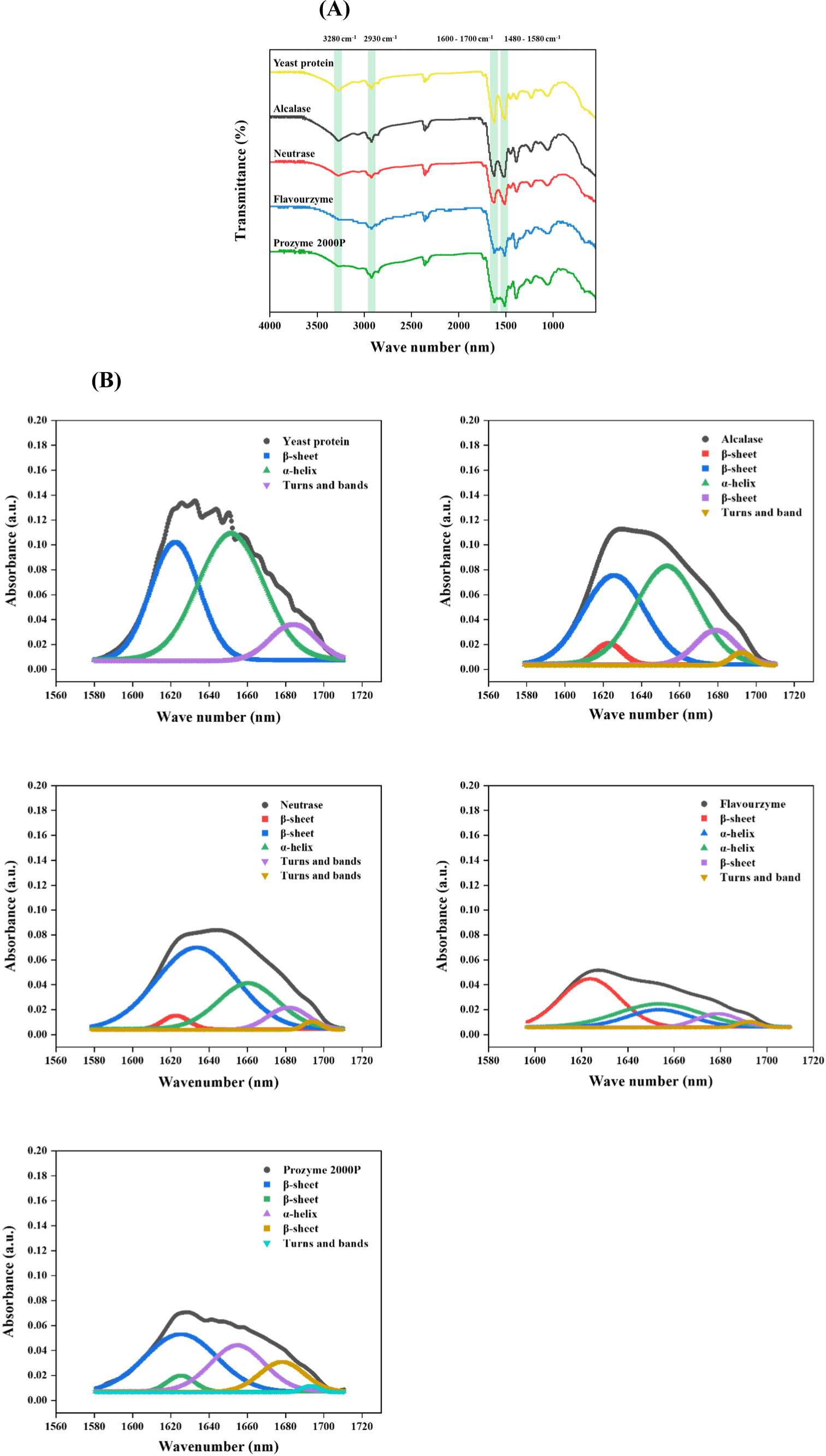
In order to clarify the changes in the secondary structure of the yeast protein, the relative proportions of secondary structures within yeast protein after enzymatic hydrolysis were investigated (Table 3 and Fig. 3B). Yeast proteins were characterized by a predominant presence of α-helix structures (i.e. about 53.30%) with 36.55% β-sheet and 10.14% β-turns. Conversely, enzymatic hydrolysis considerably altered the secondary structure of yeast proteins, exhibiting reduction in α-helix structures with β-turns, but increase in β-sheets, which shows an important feature of plant-based proteins (Carbonaro et al., 2012). The β-sheet was highly stable, whereas the α-helix and β-turn were highly flexible, exhibiting loose secondary structures (Wang et al., 2022; Xu et al., 2016). Thus, high content of the β-sheet structure provides resistance to protein breakdown in the digestive tract, which is advantageous to muscle forming (Berrazaga et al., 2019). In summary, the enzymatic hydrolysis of yeast protein increase flexibility and stability differently, but the levels may vary depending on the type of enzyme used for the treatment.
The profiles of free AAs in the yeast proteins are presented in Table 4. Yeast protein contained 313.92 mg/kg total free AAs with about 55% EAAs. Lysin is the highest amounts by following glutamic acid, implying that yeast protein can be used as an alternative to animal protein, possessing higher levels of lysine and valine than plant proteins (Day et al., 2022). After enzymatic hydrolysis of the yeast protein, the amounts of free AAs considerably increased; however, the exotype treatment showed much higher value than endotype treatment. For example, the yeast protein hydrolysates treated by exo-proteases contained over 200,000 mg/kg total AAs (TAAs) content. Among them, there are over 38,000 mg/kg aromatic AAs, above 110,000 mg/kg hydrophobic AAs, about 140,000 mg/kg EAAs. While, the yeast protein hydrolysates from endo-protease treatment showed only about 5,232–11,161 mg/kg TAAs. EAAs are indispensable in human body as they cannot be synthesized de novo or produced at a sufficient rate to meet the body’s requirements. Furthermore, dietary EAAs play a pivotal role as catalysts for skeletal muscle protein synthesis, thus holding significance in feed supplements utilized in livestock farming (Church et al., 2020). Hence, obtaining EAAs through dietary protein is imperative. Meanwhile, free AA profiles became different after hydrolysis. Interestingly, leucine was the major free AA observed in proteins regardless of enzyme types such as 1st ranked in flavourzyme (32,462.11 mg/kg), prozyme 2000P (34,292.80 mg/kg), and alcalase (1,946.93 mg/kg), and 2nd ranked in neutrase (14,413.05 mg/kg). Owing to its regulatory effects on muscle protein synthesis and lipid deposition, leucine can enhance the proportion of lean meat and reduce fat deposition, improving the feed utilization efficiency to produce high-quality pork products (Rieu et al., 2003; Zhang et al., 2020). Proteins treated with alcalase exhibit a significant generation of glutamic acid (2,341.31 mg/kg), which can contribute to enhance the flavor in alternative food and feed industry (Lipnizki, 2010). Also, yeast proteins treated with the exotype enzyme possessed considerably higher concentrations of lysine and valine than the original yeast protein. This result suggests that yeast proteins treated with exotype enzymes can be viable alternatives to animal proteins. In addition, the concentration of hydrophobic AAs including phenylalanine, leucine, isoleucine, tyrosine, tryptophan, valine, methionine, and proline (Widyarani et al., 2016) significantly increased compared with that of the control group, except for neutrase treatment. Among the treated samples, the largest increase in the concentration of hydrophobic AAs was observed for flavourzyme-treated proteins. These increased amounts of hydrophobic AAs could serve as excellent sources of antioxidants and antihypertensive agents (Khushairay et al., 2023). Although neutrase generates the least amounts of TAAs, the ratio of EAAs to TAAs was the highest in the yeast protein, reaching 71.80%. According to the ideal model proposed by the Food and Agriculture Organization and the World Health Organization, the reference value for high-quality protein should be over 40% (Li et al., 2022). Therefore, proteins treated with neutrase, flavourzyme, and prozyme 2000P were confirmed to be of high quality compared with the control group.
Conclusion
The hydrolyzed yeast protein could be utilized as a promising auxiliary protein source in livestock food in terms of their nutritional benefits. In this study, the quality of the yeast protein hydrolysates was compared after the enzymatic hydrolysis using two endotype (alcalase and neutrase) and two exotype (flavourzyme and prozyme 2000P) enzymes. The results indicated that the proteins treated with endotype enzymes exhibited higher DH and solubilities and gave more uniform PSDs than those treated with exotype enzymes. The analysis of the secondary structure of the proteins revealed a decrease in the α-helix content and an increase in the β-sheet content upon hydrolysis, indicating an improvement in structural stability, regardless of enzyme types. AA profiling also demonstrated that enzyme treatment enhanced generations of free AAs, and mostly high-quality proteins upon hydrolysis were produced. Overall, efficient processing of yeast protein through enzymatic hydrolysis could contribute to the development of sustainable and efficient alternative protein materials for food and animal feed industries.