Introduction
Genetic selection strategies to increase prolificacy in polytocous pigs resulted in increased litter size which ultimately reduced the mean birth weight of piglets within litter (Milligan et al., 2002; Quiniou et al., 2002) and led to intra-uterine growth retardation of the embryos due to limited placental capacity (Bauer et al., 1998; Stange et al., 2020). It has been shown that low birth weight (LBW) piglets (0.95–1.3 kg) exhibit a reduced post-natal growth rate and finally a lower lean carcass percentage than normal birth weight (NBW) piglets (1.4–1.6 kg) at slaughter (Bee, 2004; Gondret et al., 2006; Morise et al., 2008). The total number of muscle fibers in a muscle is an important determinant of the total mass of a muscle (Luff and Goldspink, 1970; Miller et al., 1975) because fast growing pig strains tend to have a higher number of muscle fibers in their muscles than their slow growing counterparts (Ezekwe and Martin, 1975; Miller et al., 1975). There is some evidence that LBW (runt) piglets tend to grow more slowly and less efficiently than their NBW (larger) littermates (Powell and Aberle, 1980). As a result, piglets with LBW require a longer growth period than do the NBW piglets or their heavier littermates to reach the same slaughter weight (Wolter et al., 2002) and their growth is characterized by a lower feed efficiency that ultimately results in a reduced rate of weight gain as well as a lower lean meat yield and meat quality than NBW pigs at slaughter (Gondret et al., 2005b; Rehfeldt and Kuhn, 2006). Moreover, birth weight in pigs is related to post-natal muscle development, fat accretion, and ultimately meat quality (Bee, 2004; Gondret et al., 2005a; Poore and Fowden, 2004) although contradictions exist (Bérard et al., 2008). Chemical analysis revealed that at slaughter, muscles of LBW piglets contain less intramuscular fat and protein but more water than their NBW littermates (Rehfeldt and Kuhn, 2006) and this difference was more pronounced in locomotive (semitendinosus) muscle than in postural (longissimus) muscle (Gondret et al., 2005a) although contradictions exist for intramuscular fat content (Rehfeldt et al., 2008). In practice, LBW piglets are grown by fostering or feeding them individually from birth to slaughter. This might cause the carcasses to be fatter and have increased intramuscular fat compared with their NBW counterparts (Powell and Aberle, 1980). At weaning, LBW piglets weighed 12% less than NBW piglets and required 12 more days to reach the same slaughter weight (Gondret et al., 2005a).
The total number of muscle fibers in a muscle is lower in LBW piglets than in NBW piglets or their heavier littermates, a characteristic fixed at birth (Wigmore and Stickland, 1983). Although LBW piglets have fewer muscle fibers at birth, the muscle fibers they do have are larger in mean diameter or cross-sectional area at slaughter weight (Gondret et al., 2005a; Kuhn et al., 2002) which might contribute to increased meat toughness in longissimus muscle as tenderness score and muscle fiber diameter are negatively correlated (Gondret et al., 2006), although Maltin et al. (1997) did not find any relationship between muscle fiber cross-sectional area and meat tenderness in pork. The variation in the total number of muscle fibers in a muscle in relation to piglet birth weight is not always consistent (Dwyer et al., 1993). It was shown that muscles with a lower total number of muscle fibers that have large mean diameter or cross-sectional areas are prone to rapid post-mortem pH decline and high drip losses that ultimately alter meat tenderness (Lengerken et al., 1997) and lead to pale, soft exudative pork (Rosenvold and Andersen, 2001). Muscle characteristics such as muscle fiber type composition, intramuscular fat content, total collagen content and collagen heat-solubility differ with birth weight and influence meat quality (Lebret et al., 1999).
During the fetal and early post-natal stages, the development of different tissues in the animal body is prioritized according to nutrient supply (Lawrence et al., 2012). It has been demonstrated that ovine fetuses exposed to under nutrition in utero possess more intramuscular fat in their muscles (Bispham et al., 2003; Bispham et al., 2005; Symonds et al., 2003). It was suggested that when muscle cannot form, intramuscular fat and connective tissue components increase in muscle as a default development pathway (Kablar et al., 2003). It is well accepted that LBW piglets, weighing less than 0.93 kg at birth, might exhibit slower post-natal muscle development, however they exhibit increased adipose tissue development during their lifetime (Gondret et al., 2006). It was observed that LBW piglets fed a high-fat diet were susceptible to insulin resistance (Fontaine et al., 2019). In fact, it has been shown that odd chain fatty acids (C15:0 and C17:0) from dairy fat sources are linked to a reduced risk of diabetes (Imamura et al., 2018). The addition of dairy products to the diet of LBW piglets may help to alleviate the metabolic consequences of insulin resistance in piglets. From the above discussion, we hypothesized that LBW piglets require a longer time to reach slaughter weight due to their lower growth rate and produce carcasses with lower yields of lean carcasses with inferior quality meat. This study investigated the influence of piglet birth weight on overall growth performance, carcass components and traits and muscle fiber characteristics, intramuscular fat content, collagen characteristics in the intramuscular connective tissue of longissimus thoracis muscle and the consequences for meat quality. Moreover, we would like to determine the effect of high dairy fat diet compared with high fat diet on the development of adipose tissue in LBW piglets. This was part of the other study (Wang et al., 2023) previously published.
Materials and Methods
The piglets used in this study were part of a human nutrition model study (She et al., 2022). As a result, replicate numbers were low due to the intensive nature of that research. The University of Alberta’s animal care and use committee (AUP00001184) approved both studies. According to the guidelines of the Canadian Council for Animal Care (CCAC), piglets were cared for by trained personnel.
Landrace Large White×Duroc piglets were weighed within 24 h of full-term birth and categorized as NBW (1.89±0.02 kg, n=11) and LBW (1.05±0.04 kg, n=13). Piglets were categorized as LBW [(below 95% confidence intervals (CI)] or NBW (within or above 95% CI) using CI (95%). Randomly assigned NBW piglets received a standard commercial grower-control diet (NBW-C; 1.62±0.07 kg SEM, n=5) or a high fat diet (NBW-HF; 1.73±0.09 kg SEM, n=6). The LBW piglets were randomly assigned to either a high fat diet (LBW-HF; 1.29±0.08 kg SEM, n=8) or a high fat diet with dairy sources (LBW-HFHD; 1.18±0.06 kg SEM, n=5, 3 servings of 2,000 kcal/day). Piglets were fed maternal milk until weaning (3 weeks of age) and a standard grower diet (control chow) thereafter until 5 weeks of age. All piglets were fed a transition diet for 1 week and a 6-week experimental diet for 6 weeks. A detailed description of the control chow and high fat diets has already been published (Fontaine et al., 2019). Briefly, the control diet provided 14%, 17% and 69% of energy from fat, protein and carbohydrates, respectively. In contrast, the HF diets were 46% (mainly lard), 21% and 33% (mainly fructose) with 1% cholesterol. In the HFHD diet, the serving portion of dairy products contained whole milk powder (3.25% fat, 33 g/serving; Bulk Barn, Ajax, ON, Canada), plain yogurt (10% fat, 175 mL/serving portion; Liberté, Montréal, QC, Canada) and mozzarella cheese (28% fat, 50 g/serving portion; No name®, Brampton, ON, Canada) and the diets were isocaloric. HF dairy sources were paired-fed with those on the HF diet, and daily feed intake was measured (She et al., 2022). Piglets for both NBW and LBW treatments were obtained from different dams and live weight were recorded at birth and then weekly until 12 weeks of age (slaughter age).
At 12 weeks of age, pigs were euthanized by captive bolt and slaughtered by exsanguination and eviscerated. A blow torch was used to remove the hair from the carcasses and then the carcasses were transported to the food laboratory at Agri-Food Discovery Place (University of Alberta, Edmonton, AB, Canada) within 10 min. Upon arrival at the laboratory, the carcasses were washed with cold tap water and the hot carcass weight (HCW) was recorded. The head was removed and the carcass was hung in a walk-in chiller (4°C) and allowed to cool for 24 h. After 24 h, the cold carcass weight and carcass length (from the first cervical vertebrae bone atlas to the base of the tail) were measured and recorded. The carcass was then cut into halves and both sides were dissected into primal cuts (pork shoulder, pork belly, pork loin and pork legs) following the Canadian Food Inspection Agency Meat Cuts Manual (https://inspection.canada.ca/food-labels/labelling/industry/meat-and-poultry-products/meat-cuts/pork/eng/1348682479773/1348684480749). The weights of each primal cut were recorded, and their yields calculated as percentages of the HCW. The thoracic region of the pork loin was removed from the right side of the carcass, weighed, and retained for measurement of meat quality at 24 h post-mortem. The pork loin thoracic region was fabricated for meat quality analysis as illustrated in Fig. 1. One chop (Fig. 1, steak A at 24 h post-mortem) was analyzed to measure subcutaneous back fat depth and loin muscle depth following Teixeira et al. (2021) and described elsewhere in detail (Wang et al., 2023). These data were used to calculate the Canadian Lean Yield (CLY) percentage as described by Pomar and Marcoux (2003) using the following Eq. (1):
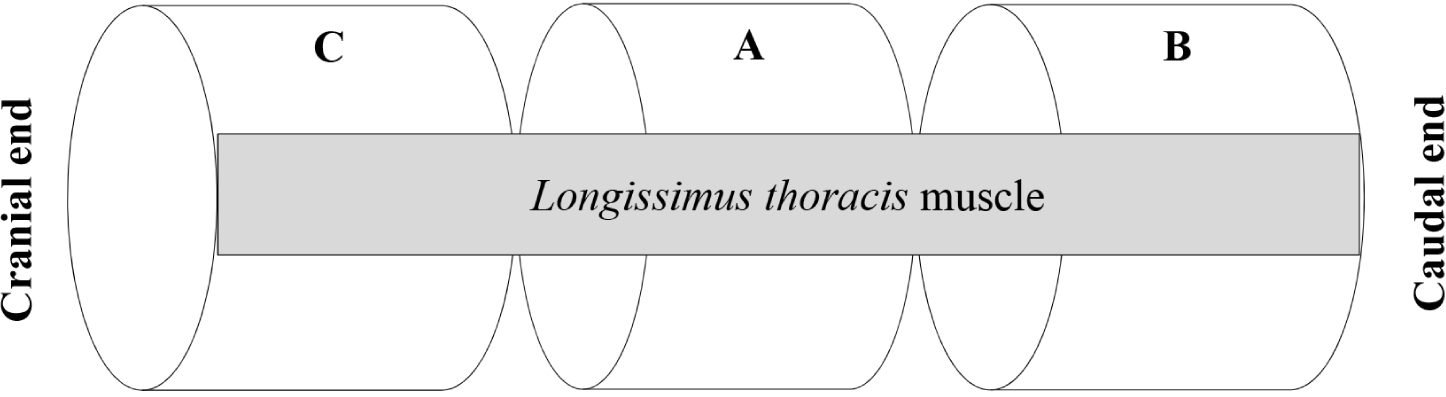
Pork meat quality characteristics were determined from chops removed from the m. longissimus thoracis (loin eye) muscle as described in Fig. 1.
Meat color was determined on a freshly cut cross section surface of a chop from the longissimus thoracis muscle after being exposed to air (bloom) for 20 min at room temperature. Surface color was measured using a CR-400 chroma meter (Konica Minolta, Osaka, Japan) with the CIE standard color system where CIE L* (ranging from 0 for black to 100 for ideal white), CIE a* (color coordinates where positive values indicate CIE a* and negative values represent greenness), CIE b* (color coordinates where positive values reflect CIE b* and negative values indicate blueness), and color intensity or saturation (C*; chroma and h*; hue) index (Commission Internationale de I’Eclairage, 1986) were recorded. The aperture area diameter was 8 mm with a 2° standard observer angle, and the D65 illumination setting was applied. The chroma meter was calibrated before the measurement using a white calibration ceramic tile provided by the manufacturer. The calibration index was used (Y=93.1, x=0.3160, y=0.3324) and the readings from the white plate were recorded (CIE L*=97.55; CIE a*=0.04; CIE b*=1.89; Chroma=1.89 and hue=88.97). Three readings were performed on each chop, and the mean of the three readings was taken for statistical analysis. Readings were performed on the lean portion of each chop, avoiding intramuscular fat and connective tissue.
An Accumet AP71 pH meter (Fisher Scientific, Mississauga, ON, Canada) was used to measure the ultimate pH at 24 h post-mortem with a pH electrode that was temperature compensated (Cat No. 655-500-30, FC210B, Canada-wide Scientific, Ottawa, ON, Canada) and calibrated with commercial pH standards 4.0 and 7.0. The glass electrode was inserted into cuts made in the muscle with a knife along with the temperature probe. The average of three readings was calculated and used for statistical analysis.
Drip loss was determined by the standard bag method according to Honikel and Hamm (1994). For this, approximately 50–60 g of longissimus thoracis muscle trimmed off epimysium was suspended in an inflated plastic bag with a metal hook for 24 h at 4°C. Drip loss was considered and calculated as the weight loss during the 24 h suspension period as a percentage of the initial muscle weight.
After color measurement, the chops were weighed, penetrated with a thermocouple (Tiny-Tag View 2S, Gemini Data Loggers, Chichester, UK) at the geometric center of the chop to monitor the cooking temperature and then grilled on an electric clam-shell style grill (General Electric 4 in 1 Grill/Griddle, Guangzhou, China) at 163°C. Chops were cooked until the temperature at the geometric center of each chop reached 71°C. At 71°C, the cooked chop was immediately removed from the grill, placed in a plastic bag, and immersed in ice water. This stop the cooking process. Once the chops cooled, the condensed fluid in the plastic bag was poured out. The chops were stored overnight in a plastic bag at 4°C. In the following day, the chops were patted dry with paper towels and weighed to determine the cooking loss using the following Eq. (2):
WBSF was measured on grilled chops stored in a cooler overnight. The grilled chops were taken out of the cooler and kept at room temperature (22°C–25°C) about 30 min for temperature equilibrium. A metal Cork borer was used to bore six 1.27 cm diameter cores with parallel to the muscle fibers direction from each cooked chop, avoiding thick visible fat or connective tissue. In order to measure peak force, a V-shaped shear blade was attached to a material testing machine (Lloyd Instrument LRX plus, AMETEK®, Digital Measurement Metrology, Brampton, ON, Canada) and cut at a cross-head speed of 200 mm/min and a pre-load force of 2 N. A mean of six peak shear force values expressed as Newtons was obtained and used for statistical analysis.
About 100 g of raw meat steak was trimmed off epimysium and cut into small cubes that were evenly distributed in a small aluminum tray. The tray covered with perforated aluminum foil, and then frozen at –20°C until lyophilized for 5–7 days. After lyophilization the weight loss was reported as the moisture content (as a percentage of total raw meat weight) and was calculated by the Eq. (3):
The crude fat content was measured with the SoxtecTM 2050 fat extraction apparatus (Foss® Analytical, Hilleroed, Denmark) following Roy et al. (2018) according to the Association of Analytical Communities Official Method of Analysis, 991.36 (Thiex et al., 2003). About 2.0 grams of lyophilized ground meat were weighed into a cellulose thimble (33×80 mm, FossTM Thimbles for SoxtecTM 2055 Manual Fat Extraction System, Fisher Scientific, Cat No. TC15220045) and packed with defatted cotton (FossTM Accessories for SoxtecTM Extraction Systems: Fisher Scientific, Catalog No.TC15290009). The extracted fat was calculated as a percentage from raw meat using the following Eq. (4):
Based on the AOAC (1990) method, crude protein was determined with a LECO FP-2000 Nitrogen Determinator (LECO, St. Joseph, MI, USA). About 100 mg of lyophilized ground meat was weighed into a foil boat liner (Cat. No. 502-343, LECO) and the weight was recorded. Standards, blanks and calibration procedures were performed with LECO TruMac N with TruMac operating software as described in the manufacturer’s operator instruction manual. Ethylenediaminetetraacetic acid (EDTA, 9.65% nitrogen, LECO) was applied as a standard for calibration after every 20 samples. Helium was used as a carrier gas, and nitrogen was measured with a thermal conductivity detector. Nitrogen was converted to protein concentration using the conversion factor of 6.25, which assumes meat protein contains 16% nitrogen.
Approximately 2.0 grams of lyophilized ground meat were weighed into dried and pre-weighed Pyrex glass bottles. The glass bottles with lyophilized ground meat were then placed into an oven for 24 h at 110°C and then into a furnace at 490°C for 24 h to incinerate the meat into ash. Then, the glass bottles with ash were cooled at room temperature in a desiccator and checked for weight. The lyophilized ground meat content after incineration into the furnace was ash and calculated as a percentage on a raw meat basis. This was done according to the following Eq. (5):
Soluble collagen was extracted from lyophilized ground meat according to the Hill (1966) method. About 2.00 grams of lyophilized meat were weighed, the weight recorded, and then the meat was heated with 18 mL ¼ Ringer’s solution at 77°C for 1 h in a 25 mL Teflon-capped glass tube. Following heating, the tubes were cooled to room temperature, and then the supernatant (soluble collagen fraction) and residue (insoluble collagen fraction) were collected following centrifugation at 3,500×g for 10 min. Extractions were performed in duplicate and means were used for statistical analysis.
An aliquot of two 1 mL of supernatants (for soluble collagen) and about 0.30 g of residue (for insoluble collagen) was hydrolyzed in 6 mL of 6M HCl for 20 h in a 20 mL glass Teflon-capped test tube. After hydrolysis, tubes were cooled in ice water to stop hydrolysis, and filtered (Whatman No. 4 filter paper, Fisher Scientific, Edmonton, AB, Canada). Filtered hydrolysates were evaporated to dryness, reconstituted in deionized water, and neutralized with NaOH. After neutralization, hydrolysates were evaporated to dryness, combined with 5 mL of deionized water, followed by the measurement of soluble and insoluble collagen content with a hydroxyproline assay.
Hydroxyproline content was determined following the Bergman and Loxley (1963) method for soluble and insoluble collagen quantification.
For hydroxyproline determination, 1.0 mL of soluble or insoluble collagen hydrolysate was obtained. The blank was prepared in the same manner as the samples using deionized water (1 mL). Absorbance was measured against the blank at 558 nm. Hydroxyproline standards (trans-4-Hydroxy-L-proline, Sigma-Aldrich) with concentrations of 2.5, 5, 10, 20 and 40 μg hydroxyproline/mL solutions were prepared. The hydroxyproline concentration in the sample was calculated using a standard curve determined by regressing the concentration of each standard against its absorbance. Multiply the concentration by the dilution factor for each sample. Hydroxyproline content was calculated based on the calculation of Stanton and Light (1987), where hydroxyproline content was converted into collagen concentration (mg/g raw meat) using a conversion factor of 7.14. Total collagen was determined by adding soluble and insoluble collagen together and collagen solubility was determined with the formula:
For each sample, duplicates were performed and their mean used for statistical analysis.
Muscle fiber typing with histological and immuno-histological characterization of the longissimus thoracis muscles was conducted by collecting 1 cm3 muscle samples from each muscle fabricated at 24 h post-mortem. Muscle cubes were immersed in acetone previously cooled with dry ice as described by Roy et al. (2018). The muscle cubes were then stored at –80°C until further processing. The muscle cubes were sectioned (10 μm thick) transverse to the muscle fiber direction in a cryostat (Leica CM1850 cryostat, Leica Biosystems Nussloch, Nußloch, Germany) at –25°C and serial sections were mounted on dry slide glasses and stored at –80°C until staining. At staining, the slide glass with mounted muscle sections was removed from storage and air-dried at room temperature for 30 min. Muscle fiber typing was performed using the myofibrillar adenosine triphosphatase (mATPase) staining method (Brooke and Kaiser, 1970) and the nicotinamide adenine dinucleotide dehydrogenase‐tetrazolium reductase (NADH-TR) staining method (Roy et al., 2018). Confirmation of type I muscle fiber type was performed by immuno-fluorescence histochemistry using monoclonal antibodies (S58, skeletal muscle myosin antibody from Santa Cruz Biotechnology) specific for the type I myosin isoform (Roy et al., 2018). Myofibrillar adenosine triphosphatase (mATPase) staining was done on muscle sections after pre-incubation in acid (pH 4.3) and alkali (pH 10.5). The classification of muscle fiber typing was carried out as presented in Fig. 2 by following the different staining procedures in serial sections as mentioned above. Using the open-source software ImageJ (http://rsbweb.nih.gov/ij/), cross-sectional areas of muscle fibers were measured from three randomly captured images at 200× magnification, which included at least 300 muscle fibers from each sample and were converted to diameter. To determine the muscle fiber diameter class interval relative to frequency percentages, different class intervals of the diameter (10–20, 21–30, 31–40, 41–50, 51–60, 61–70, 71–80 and 81–90 μm) of total muscle fibers counted for diameter from each treatment group were considered.
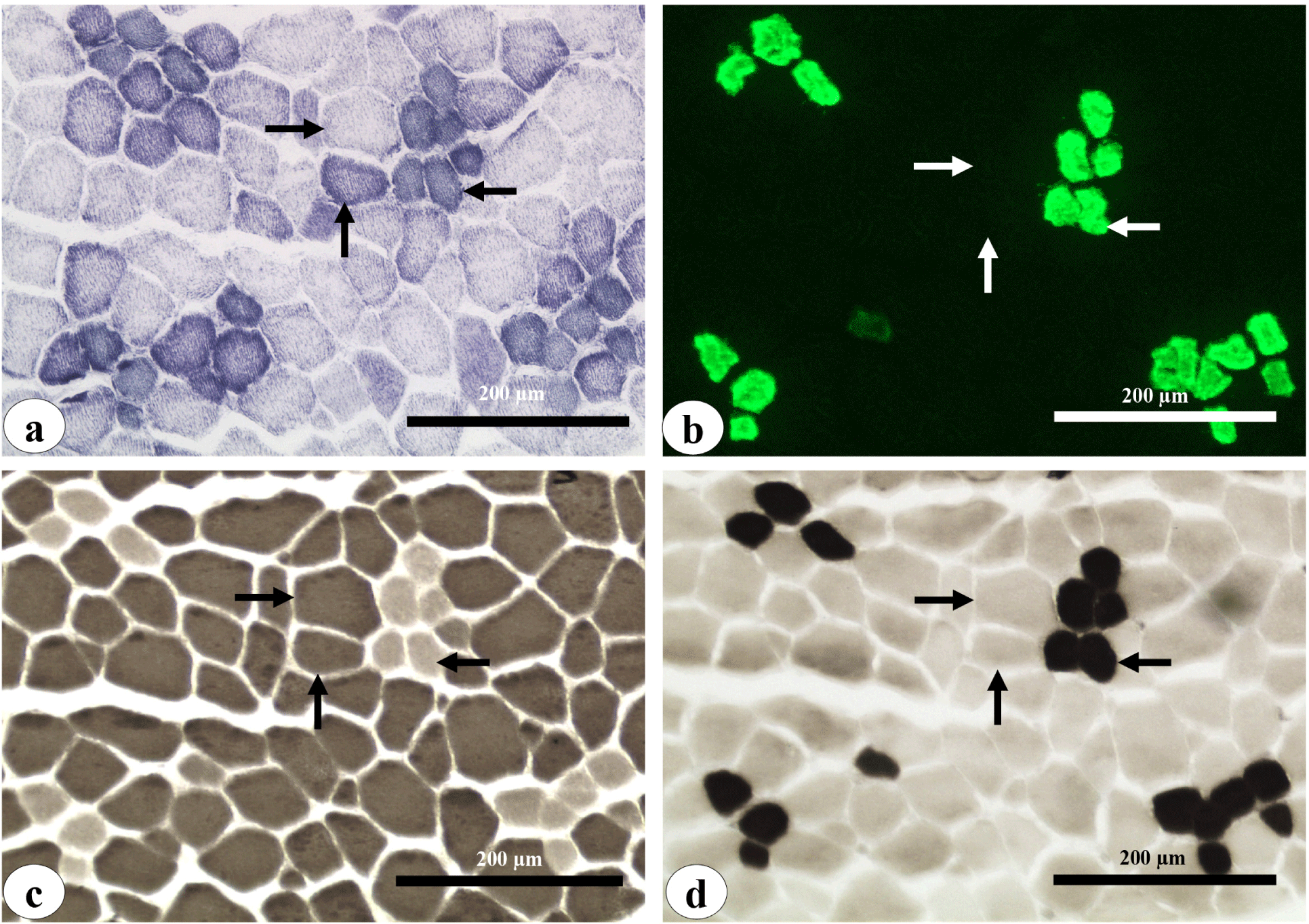
Data were analyzed by R (version 3.6.1) with the package lessR using one-way analysis of variance (ANOVA) where treatments (birth weight) were regarded as the sole source of variation. The Tukey’s Honestly Significant Difference (HSD) test was used to detect significant differences between means when significant main effects were detected. All comparisons with p<0.05 were considered statistically significant and 0.05<p<0.10 were considered to approach significance. Muscle fiber diameter class interval frequency percentages were analyzed by one-way ANOVA within each diameter interval class using piglets’ birth weight groups as the sole source of variation. Where the model was significant (p<0.05), Tukey’s HSD test was used to determine mean differences between the treatments. Pearson correlation analysis was conducted to analyze the relationship between different measurements. The correlation coefficients were calculated using the package corrplot in R (version 3.6.2).
Results
The weekly mean live weights of the piglets by treatment are presented in Table 1. As planned, the birth weight of the piglets was significantly higher (p=0.0003) in both NBW treatments (NBW-C and NBW-HF) compared with both LBW treatments (LBW-HF and LBW-HFHD) for the first 4 weeks of life. The NBW-HF pigs had heavier mean live weights than the LBW-HF and LBW-HFHD pigs up to 4 weeks of age, but by 6 weeks of age, mean live weights across the treatments were no longer different (p>0.05). From 6 to 12 weeks, the difference in live weight between NBW-HF and LBW-HFHD pigs approached significance at 8, 9, 10 and 12 weeks (0.05<p0.1; Table 1). LBW pigs fed high fat diets (LBW-HF) had mean live weights similar to that of NBW-C pigs throughout (Table 1), while LBW-HFHD pigs had mean live weights similar to that of NBW-C and LBW-HF pigs throughout as well (Table 1).
a,b Different letters in the same row are significantly different in different animal groups at the 0.05 level of probability (p<0.05).
The carcass composition of the pigs at slaughter from the different treatments is presented in Table 2. HCWs were not different between the treatments, with the difference between the normal (NBW-HF) pigs and the low (LBW-HFHD) birth weight pigs approaching significance (p=0.0615). Cold carcass weights were not different due to treatment, nor was carcass length, longissimus thoracis muscle depth and area, dressing percentage, pork shoulder weight percentage, pork loin weight percentage or pork belly weight percentage (Table 2). Mean subcutaneous fat depth was lower in the LBW-HFHD pigs than in the NBW-HF and LBW-HF pigs but did not differ from the NBW-C pigs which indicated that inclusion of dairy fat as dietary fat source in the high fat diet of pigs significantly decreased subcutaneous fat depth (p=0.0229). The pork leg proportion was significantly higher in LBW-HFHD than in NBW-C pigs but did not differ from pigs fed the other HF diets (LBW-HF and NBW-HF). The mean CLY percentage was significantly higher in LBW-HFHD pigs compared with pigs receiving other HF diets regardless of birth weight but not different from that of NBW-C pigs.
a–c Different letters in the same row are significantly different in different animal groups at the 0.05 level of probability (p<0.05).
Meat quality characteristics in longissimus thoracis muscle are presented in Table 3. There were no significant differences in the color parameters CIE L*, CIE a*, chroma, hue, ultimate pH at 24 h, cooking loss, and WBSF of the meat due to treatment. Differences that approached significance included CIE b*, with pork from LBW-HFHD pigs tending to have a higher CIE b* than that of NBW-C pork although it did not differ from pork from the NBW-HF and LBW-HF pigs (p=0.0587). Also, drip loss tended to be higher for pork from NBW-HF pigs than NBW-C pigs but did not differ from LBW-HF and LBW-HDHF groups (p=0.0985).
Both the proximate composition and collagen content of pork were determined in longissimus thoracis muscle and results are presented in Table 4. There were no significant differences between birth weight treatments for muscle crude protein, intramuscular fat, ash, total collagen, soluble collagen, insoluble collagen content and collagen solubility percentage. Moisture content was significantly higher in pork from NBW-C pigs than NBW-HF and LBW-HF pigs but did not differ from that of LBW-HDHF pigs. The mean percentage of soluble collagen in pork from NBW-C pigs tended to be higher than that of NBW-HF pigs, with this difference approaching significance (p=0.06).
a,b Different letters in the same row are significantly different in different animal groups at the 0.05 level of probability (p<0.05).
Muscle histological cross-sections depicting the three muscle fiber types (I, IIA, and IIB) from pigs of different birth weight treatments are illustrated in Fig. 2 and their properties are presented in Table 5. The total muscle fiber number in the whole cross-sectional area of the longissimus thoracis muscle was not significantly altered due to treatment, nor was the percentage of different muscle fiber types. LBW-HFHD pigs had a significantly increased mean muscle fiber diameter compared to NBW piglets receiving either control (NBW-C) or HF (NBW-HF) diets. The LBW-HFHD pig muscle showed a larger mean diameter of type 1 muscle fibers than NBW-HF pigs but did not differ from NBW-C and LBW-HF pigs. There was no difference in type IIA and IIB muscle fiber characteristics due to treatment.
The frequency distributions of type I, IIA, IIB and mean muscle fiber diameters across the different class intervals are presented in Fig. 3. Type I muscle fiber diameter in the 31–40 μm class interval tended to be more frequent in NBW pigs than in LBW-HFHD pigs (p=0.0564). The mean muscle fiber diameter in the class interval 11–20 μm appeared to be significantly more prevalent overall (p=0.0176) and type IIB muscle fibers (p=0.0211) in the NBW-HF pig muscle compared with that of the LBW-HFHD pigs. Also, the mean muscle fiber with diameters between 61–70 μm tended to be more prevalent (p=0.0741) in LBW-HFHD and NBW-C pig muscles than in NBW-HF pig muscles (0.05<p<0.1).
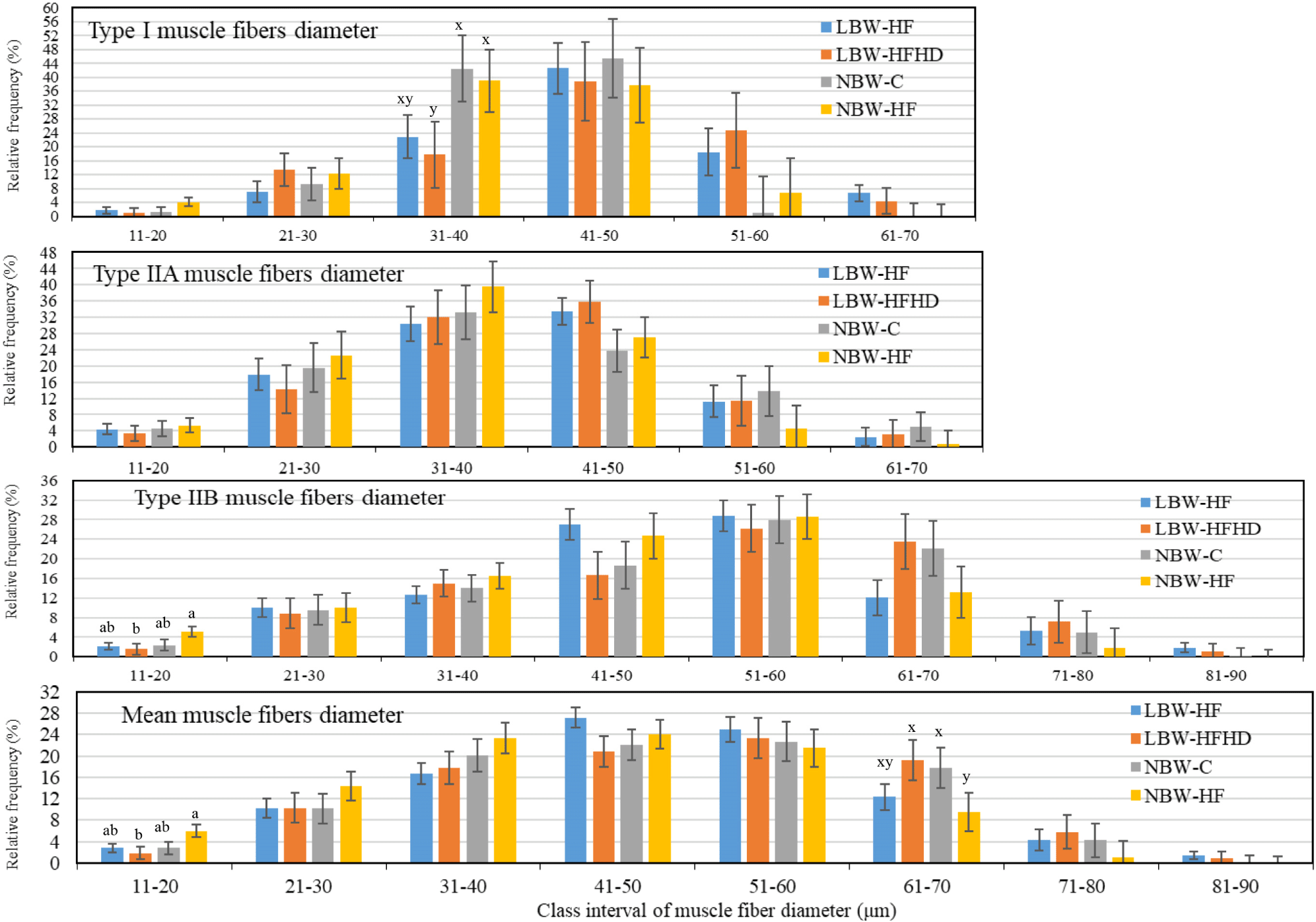
Discussion
The implications of piglet birth weight on subsequent carcass characteristics have been a major subject of pig research in the past (Alvarenga et al., 2012; Bee et al., 2007; Bérard et al., 2010; Rehfeldt and Kuhn, 2006; Rehfeldt et al., 2008). The pork industry has increased swine litter size through genetic selection and has introduced highly prolific dam lines for commercial production that result in LBW piglets due to crowding in the dam’s uterus (Quiniou et al., 2002). Also, due to the limited capacity of the uterus, the increased number of fetuses results in a reduced nutrient supply per fetus during gestation (Père and Etienne, 2000), hence LBW piglets are associated with a reduced growth rate during their lifetime (Quiniou et al., 2002; Rehfeldt et al., 2008; Zhang et al., 2018). The effect of a nutrient deficit diet during gestation on the myogenesis of a fetus is strongly influenced by the time point in gestation at which the deficiency occurs. Nutrient deficiency during the early stages of pregnancy (35–60 days of gestation) hinders the development of primary muscle fibers but deprivation during late gestation (55–90 days of gestation; Kalbe et al., 2017; Lefaucheur et al., 1995) reduces muscle fiber diameter (Greenwood et al., 1999). Consequently, nutrient deficiency at the early prenatal stage results in a lower total number of muscle fibers, altered composition of muscle fiber type, and ultimately a reduction in overall muscle mass (Ward and Stickland, 1991; Yates et al., 2012). During myogenesis, nutrient-deficient, growth-restricted fetuses reduce primary muscle fiber diameter (Wigmore and Stickland, 1983). The smaller diameter of primary muscle fibers presents a smaller surface area to serve as a scaffold for secondary muscle fibers to attach. This limits the number of viable secondary muscle fibers during myogenesis (Wigmore and Stickland, 1983). The reduction in primary muscle fiber numbers not only limits muscle growth, but also hampers formation of secondary fibers, which reduces the percentage of fast-twitch myofibers that will take on a glycolytic phenotype at maturity and results in an increase in a more oxidative phenotype in the muscle in LBW neonates (Lefaucheur et al., 2003; Wank et al., 2000). For growth, oxidative muscle fibers (Type I) exhibit greater rates of protein synthesis than glycolytic muscle fibers (Type IIA and IIB) and prefer to store energy as fat (Bates and Millward, 1983; Kelly et al., 1984; Laurent et al., 1978) which corroborate with the present study that the diameter of type I muscle fibers is larger in LBW-HFHD than NBW-C and NBW-HF ultimately help to catch-up the live weight of NBW piglets.
The results presented in this report disagreed with results from previous studies that showed that LBW piglets (Gondret et al., 2005a; Wolter et al., 2002) or lighter piglets at weaning (Gondret et al., 2005a; Mahan and Lepine, 1991; Wolter and Ellis, 2001) required more days compared to their heavier littermates to attain the same market weight. LBW neonates do not always remain smaller than their littermates throughout their growing period (Crume et al., 2014), and sometimes LBW piglets exhibit compensatory growth postnatally (Douglas et al., 2013; Rehfeldt and Kuhn, 2006; Rutherford et al., 2013). Consistent with previous studies (Bérard et al., 2008; Bérard et al., 2010), LBW piglets in the present study exhibited compensatory growth as differences in live weight between the different treatments disappeared by 6 weeks of age. LBW-HFHD pigs showed no difference in mean live weights with NBW-C and LBW-HF pigs at weeks 8, 9, 10 and 12. This is a small study, with limited replication. The differences observed from week 5 onward most likely would be significant in a study with increased replication. Differences between the treatments for live weight approached significance (0.05<p<0.1) at 5, 8, and 12 weeks, and would have been significant at 80% power and 95% confidence at a replication of greater than or equal to 8, 9 and 10, respectively.
Gondret et al. (2006) did not observe any significant differences in HCW and dressing percentages between NBW (1.89±0.02 kg) and LBW (1.05±0.04 kg) piglets when housed individually, and this was consistent with the results of the present study. Gondret et al. (2005a) speculated that LBW (0.80–1.10 kg) piglets grow slower than their NBW (1.75–2.05 kg) counterparts when placed in collective pens because LBW piglets compete less effectively for feed during the immediate post-weaning period. In this study, the piglets were individually penned and fed hence the LBW piglets had unfettered access to feed and showed compensatory growth relative to the NBW piglets which accounts for no differences due to diet being observed in HCW and dressing percentages. Other studies (Beaulieu et al., 2010; Gondret et al., 2006) did not find any differences in lean meat yield percentages between NBW and LBW pigs, whereas the present study showed that CLY percentage was higher in LBW-HFHD pig carcasses than in those from LBW-HF and NBW-HF pigs but did not differ from NBW-C pigs. Again, HCW differences were observed in the present study that may have been significant if replication was increased to greater than that used in the current study, but with the current replication complement, piglet birth weight had no significant effect on CLY percentage and loin muscle area in the present study, and this agreed with the results from previous reports (Bee, 2004; Bérard et al., 2008).
Heyer et al. (2004) reported that the percentage of primal pork cuts on an HCW basis increased. Subcutaneous (back) fat thickness decreased in NBW piglets compared to their LBW counterparts. Supplying dietary fat from dairy sources disrupted that relationship, decreasing the subcutaneous fat thickness of carcasses from the LBW-HFHD pigs compared to those from the LBW-HF and NBW-HF pigs. This may have been associated with dairy fat being the major source of energy in the diet. Gondret et al. (2006) observed that shoulder weight percentages did not differ between LBW and NBW piglets. However, but backfat depth and belly percentage were higher in LBW pigs and ham (leg weight) and loin weight were lower in LBW pigs. LBW pigs in previous studies had significantly higher intramuscular fat content as a result of being offered ad-libitum diets during growth (Kuhn et al., 2002; Poore and Fowden, 2004) or adjusted daily feed allowances during the rearing period (Bee, 2004) compared to pigs in the present study. It was reported that this compensatory growth may lead to extra fat deposition in muscle (Cho and Suh, 2016; Ibáñez et al., 2011) which was absent in the present study. Beaulieu et al. (2010) did not observe any significant effect of piglet birth weight on intramuscular fat content, which agrees with the present study results. There was no significant difference in total intramuscular fat content in the longissimus thoracis muscle among birth weight groups similar to Gondret et al. (2005a), although they observed a 25% increase in intramuscular fat content in the semitendinosus muscle of LBW pigs.
Effects on meat quality were limited, and how representative or applicable these results would be at commercial slaughter weight was not a hypothesis tested in the current study. We did not find any difference in ultimate pH at 24 h postmortem due to treatment, which contradicted the results of Gondret et al. (2006) who observed higher pH in longissimus muscle of LBW piglets compared with heavy birth weight piglets but found no difference in semitendinosus muscle though the slaughter age and weight of the LBW pigs were 153.9±2.3 days and 101.5±0.6 kg, respectively and LBW pigs required 12 days more to reach the same slaughter weight with their heavy birth weight counterparts. In some previous studies, it was observed that birth weight does not have a significant effect on drip loss and cooking loss (Beaulieu et al., 2010; Rekiel et al., 2014) in agreement with the present results although there are contradictory results from Bee (2004) and Gondret et al. (2005b) found that LBW pork meat had a higher drip loss than NBW piglets. There are reports that demonstrate that LBW piglets have fewer muscle fibers with larger diameters (Gondret et al., 2005b; Hegarty and Allen, 1978; Powell and Aberle, 1981) and Lengerken et al. (1997) claimed that meat from these muscles showed increased drip loss and lower ultimate pH which are generally associated with increased meat toughness (Minelli et al., 1995; Monin et al., 1999).
Beaulieu et al. (2010) observed that birth weight differences in piglets had no significant effect on the color characteristics (CIE L*, CIE a*, CIE b*, chroma and hue values) of the meat, and this result agreed with the present study results. There are other studies that observed increased CIE a* (higher CIE a*; Bérard et al., 2008; Rekiel et al., 2014) and increased CIE L* (higher CIE L*) when measured at 1-day post-mortem (Gondret et al., 2006) in LBW piglets’ longissimus muscles. The difference in pork color properties in the previous studies may be due to measurements conducted at different times post-mortem. Choi and Oh (2016) observed that pigs with heavier carcass weights had lower CIE a* and ultimate pH at 24 h in longissimus muscle compared to pigs with lighter carcass weights. They did not observe any difference between these two carcass groups in CIE L* and CIE b*. According to Choi and Oh (2016) the higher growth rate associated with heavy carcasses produced larger muscle fiber cross-sectional areas in both types IIA and IIB muscle fibers and muscles had lower ultimate pH values at 24 h post-mortem.
In the present study, the loin WBSF did not show any significant differences between birth weight groups, which was inconsistent with the results of Gondret et al. (2005a) who concluded that LBW piglets produced less tender meat. Gondret et al. (2005a) found that mean muscle fiber diameter was negatively correlated with tenderness and that LBW piglets had a larger mean diameter than NBW pigs. In the present study, the WBSF of longissimus thoracis muscle did not differ between LBW and NBW treatments regardless of dietary fat supplementation. This agrees with Bérard et al. (2008) who did not find any differences in WBSF of cooked meat from longissimus muscle.
It is now an established idea that the number of total muscle fibers in a muscle is fixed before birth in pigs (Wigmore and Stickland, 1983) although contradiction exists as Mascarello et al. (1992) reported that proliferation of muscle fibers also occurs in the neonatal period. The total muscle fibers number in the whole cross-sectional area of the longissimus thoracis muscle was not significantly different due to treatment in the present study. There is evidence that hypertrophy of individual muscle fibers is higher in the post-natal period in muscles with a lower number of muscle fibers (Hegarty and Allen, 1978; Rehfeldt et al., 2000); therefore, differences in muscle fiber characteristics between LBW and NBW pigs were expected. Indeed, previous studies have shown that the mean muscle fiber diameter in the semitendinosus and longissimus muscles of pigs was higher in LBW compared with NBW at the same market BW (Bee, 2004; Gondret et al., 2005a; Handel and Stickland, 1987) or at the same age (Kuhn et al., 2002). In pig muscle, larger diameter muscle fibers and or a reduced total number of muscle fibers have been suggested to lead to a lower pH (Lengerken et al., 1997). The larger mean diameter of muscle fibers in longissimus thoracis muscle of LBW-HFHD piglets compared to NBW-HF agreed with other studies (Gondret et al., 2005b; Hegarty and Allen, 1978; Powell and Aberle, 1981) who found that muscles with a lower number of muscle fibers compensate growth through increased muscle fiber size. Rehfeldt et al. (2000) suggested that hypertrophy of muscle fibers might compensate for the decrease in muscle fiber number. In the present study, the total number of muscle fibers in the cross-sectional area of longissimus thoracis muscle did not differ significantly between birth weight treatments, and this result agrees with other studies (Dwyer et al., 1993; Handel and Stickland, 1987). There is contradictory evidence of LBW piglets having a lower total number of muscle fibers in longissimus muscle than NBW piglets (Dwyer and Stickland, 1991; Gondret et al., 2005b; Rehfeldt et al., 2004; Wigmore and Stickland, 1983). It was observed in previous studies (Beaulieu et al., 2010; Bee, 2004; Gondret et al., 2006; Rehfeldt et al., 2008; Wigmore and Stickland, 1983) that LBW (around 1 kg) piglet muscle had a larger mean diameter for slow-twitch-oxidative (type I) muscle fibers similar to the present study. These studies also did not observe any adverse effect on meat quality with an increased mean diameter of type I muscle fibers. This agrees with the present study results. The results of this study confirmed that skeletal muscle mass is determined by the total number of muscle fibers and their cross-sectional areas (Dwyer et al., 1993). It has been reported that small pig fetuses have a reduced number of muscle fibers (Wigmore and Stickland, 1983) but these authors did not confirm that this persisted at slaughter. Moreover, it was shown that muscle fiber type composition is not always correlated with live weight at the time of slaughter, so carcass weight is often used for comparison (Jeong et al., 2012). It has been reported that piglet birth weight has no significant effect on muscle fiber type composition at slaughter weight of pigs (Bee, 2004; Gondret et al., 2005a; Rehfeldt and Kuhn, 2006), and the results of the present study agreed. Gondret et al. (2005a) explained that muscle fiber orientation in longissimus muscle is not parallel to the longitudinal direction, making the estimation of total muscle fibers counts crucial or relevant for this muscle. Also, skeletal muscle size is determined not just by the number of total muscle fibers it contains, but also by their combined variable cross-sectional area and length (Dwyer et al., 1993).
Gondret et al. (2006) observed increased insoluble collagen and a tendency for higher total collagen content in the longissimus thoracis muscle of LBW pigs compared to NBW pigs. However, there was no difference in collagen heat solubility percentages. Further, these authors did not observe any difference in collagen characteristics in the semitendinosus muscle regardless of birth weight. Clelland (2001) however found that semitendinosus muscle from LBW piglets in the same litter had a higher proportion of intramuscular connective tissue and Karunaratne et al. (2005) found an increase in collagen content associated with LBW. The present study confirmed that intramuscular collagen content and intramuscular fat were not affected by birth weight. This is an indication that the longissimus muscle may be less sensitive to the implications of birth weight than the semitendinosus muscle. It might also be that collagen content has no significant effect on the toughness of pork from young pigs weighing less than 100 kg liveweight at slaughter (Kirkegaard et al., 1979). It is well established that muscle from LBW piglets contains more intramuscular fat and less lean muscle than NBW piglets (Bee, 2004; Gondret et al., 2005a; Poore and Fowden, 2004) which ultimately indicates that LBW piglets are prone to increased non-muscle components in their semitendinosus muscle mainly collagen type I and intramuscular fat at 86 days of gestation (Karunaratne et al., 2005). Intramuscular fat and connective tissue cells are the default differentiation pathway when muscle cells cannot form during gestation or the early post-natal period (Kablar et al., 2003). Increased intramuscular fat contents are due to larger mean adipocyte diameter in the skeletal muscle of LBW piglets than in NBW piglets (Powell and Aberle, 1981) although in the present study there was no observed difference in intramuscular fat content. When LBW pigs were fed the high fat diet, intramuscular fat contents increased in LBW pigs compared with NBW pigs (Liu et al., 2014). Other studies indicated that intramuscular fat content was similar among different birth weight pigs at slaughter (Gondret et al., 2005a; Powell and Aberle, 1980; Wolter et al., 2002) and this is consistent with the present study. Compared to NBW piglets, LBW piglets had lower subcutaneous fat depth when slaughtered at the same live weight (Rekiel et al., 2015), which contradicted the present study results, although the LBW-HFHD piglets showed decreased subcutaneous fat depth. Collagen content and intramuscular fat in muscle are important characteristics for the meat industry. An increased amount of collagen may increase meat toughness while higher intramuscular fat may improve meat tenderness (Fang et al., 1999; Gondret et al., 2005a). Discrepancies on the effects of birth weight with previous studies on live weight, carcass parameters, physical properties of meat, chemical composition (proximate and collagen content) probably arise from differences in genotype, birth weight delineation, nutrient composition in the diets, feeding level and fat content and sources of fat in the diet.
Some earlier studies showed that an increase in the mean diameter of muscle fibers has a negative influence on meat quality (Carpenter et al., 1963; Karlsson et al., 1993; Maltin et al., 1997) specifically increasing WBSF and drip loss (Minelli et al., 1995; Monin et al., 1999) but in the present study we did not observe any significant correlation between mean muscle fiber diameter with WBSF (r=–0.14) and drip loss (r=–0.34). Although there was no effect of birth weight or dietary treatment on meat quality in this study, dietary fat from dairy sources reduced subcutaneous fat depth and increased CLY percentage in LBW piglets that received a diet high in fat from dairy sources (LBW-HDHF) without compromising longissimus muscle depth. Rehfeldt and Kuhn (2006) speculate that lower muscle fiber numbers in LBW piglets result in maximum hypertrophy in muscle fibers before reach to slaughter weight. This might divert available dietary nutrients towards fat storage instead of muscle accretion. LBW pigs have been reported to produce carcasses with excessive fat (Bérard et al., 2010) due to the ratio of protein and energy in diets suitable which is suitable for NBW pigs, but more energy is available for fat deposition in LBW pig muscle (Kerr et al., 2003; Wang et al., 2018). How the source of dietary fat could cause this is unknown, although dairy fat has a high proportion of saturated fats, which have been linked with high satiety (Kozimor et al., 2013). Milk saturated fats have a high proportion of medium chain triacylglycerols (Ruiz-Sala et al., 1996), which are linked to satiety and lower caloric intake in men (St-Onge et al., 2003). Wang et al. (2023) reported that the feed intake of the LBW-HFHD pigs was in fact lower than that of pigs receiving the other diets, confirming that dairy fats decreased feed intake. The inclusion of dairy fats in LBW pig diets may balance feed intake with protein synthesis capacity. This may decrease the amount of energy derived from the diet, reducing carcass fat and increasing lean meat yield. Further investigation of the impact of dietary fat sources on LBW pig growth and carcass quality is warranted in a larger study.
Conclusion
This preliminary study confirmed that LBW piglets exhibit compensatory growth and that growth is associated with hypertrophy of slow-twitch-oxidative (type I) muscle fibers and reflected by mean muscle fiber diameter which is larger in LBW groups at slaughter. Effects of birth weight on meat quality were limited, however, indicating that the pork quality is not compromised by LBW. Further studies should focus on modifying carcass composition, including decreased back fat thickness in LBW piglets on dairy-sourced high-fat diets.