Introduction
Because freezing involves in phase conversion from water to ice, freezing is progressed by a three-stage mechanism including cooling of water (pre-cooling), phase transition (primary freezing) and cooling of the ice (secondary freezing). During the pre-cooling stage, water is supercooled, which is a state in which water is not converted to ice even at sub-zero temperatures. Once ice seeds (namely nuclei) are formed (nucleation), water begins to be converted to ice (phase transition). Therefore, the number of ice crystals is closely related with the extent of supercooling (Fernández et al., 2006). When the ice nuclei are formed, the temperature of water increases to its freezing point due to release of heat of fusion, and thereafter ice crystal is grown. The size of ice crystals is proportional to duration of phase transition. Because the number and size of ice crystals affect the degree of tissue damage, the ideal freezing is supposed to be large supercooling with short phase transition time (Otero and Sanz, 2006).
Frozen meat is vulnerable to drip loss during thawing, hence the ideal quick freezing is essential to prevent quality loss. However, the supercooling phenomenon is rarely regulated due to its metastable nature, commercially adopted quick freezing techniques focus on rapid phase transition by supplying a very low air temperature or force air convection system. However, these freezing techniques are based on heat transfer from the inside to the outside through body, and the freezing occurs from the surface to the center of the materials. Therefore, the surface of meat can be frozen quickly, whereas the center position of the meat experiences slow freezing, even though a rapid freezing is applied (Sanz et al., 1999).
Contrarily, up to date freezing technologies are based on the application of artificial supercooling prior to secondary freezing. Under hydrostatic pressure or electromagnetic fields, for instance, meat can be held in a supercooled state under sub-zero temperature (Luscher et al., 2005; Otero and Sanz, 2000). The supercooled meat is frozen instantaneously irrespective of the meat position by releasing pressure or electromagnetic fields. Still, these techniques involve in problems such as pressure-induced protein denaturation (Fernández et al., 2000) or extremely high electromagnetic field requirements (Otero et al., 2016). Alternate simple way to generate artificial supercooling is to cool down the meat very slowly (Chen and Lee, 1998; Tan et al., 2016). However, the slow cooling also leads to overall slow freezing of meat, which limits the application for meat freezing.
In this study, it was hypothesized that supercooled meat would be frozen quickly compared to that without supercooling, which would prevent or reduce the quality deterioration of frozen meat. Since the nucleation is easily detected by monitoring the temperature of meat, a point at which nucleation occurs may be equivalent with the maximum extent of supercooling. Subsequently, the meat in which nucleation occurs is easily cooled down during secondary freezing even if slow freezing is applied. To confirm this hypothesis, it is essential that pre-cooling and freezing should be applied under different conditions (different temperature or cooling rate). Consequently, the present study investigated the effect of artificially produced supercooling followed by air convection freezing (normally recognized as slow freezing) on the quality characteristics of pork.
Materials and Methods
Seven pork loins (m. longissimus dorsi) with normal post-rigor pH (pH 5.4-5.7) were purchased at 24 h postmortem from a local market. Four rectangular forms (2 × 3 × 8 cm) parallel to the direction of muscle fiber were sampled from the inside of each loin. After weighing all samples, a thermocouple (k-type, alloy combination of Ni-Cr and Ni-Al) was inserted into the geometric center of each sample, while the other side of the thermocouple was passed through the package and sealed with a gluegun for vacuum-packaging. All vacuum-packaged samples were tempered in a refrigerator until the core temperature reached at 4℃.
Temperature profiles of pork during freezing were monitored by connecting the thermocouple to a data logger (MV104, Yokogawa Co., Japan). The samples from each of the seven loins were divided by 4 groups (total 7 samples per treatment). The first group was kept at 4℃ for 24 h and used as the unfrozen control (CT). The second group was frozen in a -18℃ conventional freezer (still air type for slow freezing, SAF) and the initial freezing point of the sample (-0.6℃) was monitored. The third group was immersed into -30℃ ethanol (EIF) for rapid freezing. When the core temperature of the meat was reached at -18℃, the meat samples were moved to the -18℃ freezer. The last group was subjected to artificial supercooling followed by still air freezing (SSF). To generate artificial supercooling, the meat samples were slowly cooled down to sub-zero temperature (Chen and Lee, 1998). For slow cooling, the meat was immersed into a 0℃ cryostat and tempered. When the core temperature of the sample reached to 0℃, the temperature of the cryostat was decreased down to -0.5℃ and tempered. This process was repeated with decreasing the temperature of cryostat by 0.5℃ units (minimum unit of device) until the core temperature was increased to its initial freezing point (-0.6℃, nucleation was occurred). After the nucleation was detected, the sample was removed from the cryostat and moved to the -18℃ freezer for the final freezing process. All frozen samples were kept at -18℃ for 24 h. After storage, one sample from each treatment was randomly selected and lyophilized to observe microstructure. The remaining all samples were thawed in a running water for 15 min (~3℃ of core temperature) to diminish any thawing effects, and weighed to determine thawing loss. Two samples of each treatment were used to determine expressible moisture and color, while the remaining four samples were cooked to evaluate cooking loss and shear force. This whole procedure of the experiment was repeated three times on different days for experimental replications (n=3).
The freezing rate of all treatments was calculated by half thickness of the sample (1 cm) per time traversing from initial freezing point (-0.6℃) to -5℃ (Heldman and Taylor, 1997). The extent of supercooling (ΔT) of the SSF treatment was defined by temperature difference of the freezing point of pork and the nucleation temperature.
Immediately after thawing, the surface exudates of the meat samples were gently wiped off using tissue, and all thawed meat samples were weighed. Thawing loss of frozen pork was estimated by percentage weight loss over its initial weight. For the control, drip loss generated for 24 h in a 4℃ refrigerator was estimated and regarded as thawing loss of control.
Two samples from each treatment were randomly selected, and approximately 1 g of sample was taken and moved to a centrifuge tube along with a gauze as an absorbent. The tubes were centrifuged at 1,500 g for 15 min, and the meat pellet was carefully removed. The tubes were weighed and dried at 102℃ overnight. The dried tubes were weighed again and expressible moisture was determined by the percentage amount of the released moisture over the initial sample weight (Trout, 1988).
Thawed meat samples were kept in ambient air for 10 min, and the surface color of the sample was taken using a color reader (CR-10, Konica-Minolta Sensing Inc., Japan) calibrated with a standard white board (L* = 97.8, a* = -0.4, b* = 2.0). CIE L*, a* and b* were measured as indicators of lightness, redness, and yellowness, respectively (Schanda, 2007).
Four samples from each treatment were cooked in a 75℃ water bath for 15 min and tempered for 30 min at ambient temperature. The weights of cooked samples were measured, and cooking loss was calculated by percent weight loss over its weight after thawing based on Honikel (1987).
Shear force of each treatment was measured by the method of Barbanti and Pasquini (2005) with minor modification. The cylindrical sample parallel to the muscle fiber was taken from the center of the cooked samples using a 10 mm cork borer. The shear force was measured using a texture analyzer (CT3, Brookfield Engineering Labs Inc., USA) equipped with a knife (TA-SBA, Brookfield Engineering Labs Inc., USA). Meat samples were sheared under the condition of 1 mm/s test speed and 1 g of trigger load.
The frozen samples were directly lyophilized at 0.6 Torr for 48 h. The dried meat was cut with parallel and vertical to the direction of muscle fiber. The meat piece was mounted on the sample holder and gold-coated for 5 min using an ion sputter (E-1010, Hitachi science system Ldt., Japan). The microstructures of the samples were observed using a scanning electron microscope (S-3000N, Hitachi science system Ltd., Japan) at 20 kV of the accelerating voltage and ×100 of magnification.
Results and Discussion
Pork did not show a supercooling phenomenon (SAF and EIF), unless supercooling was artificially conducted (SSF) (Fig. 1). To obtain artificial supercooling, the cooling rate of SSF had to be regulated slower (0.13℃/min) than that of SAF (0.20℃/min) by which a limited extent of supercooling (ΔT = 1.4℃) was obtained. Nevertheless, freezing profiles of pork demonstrated that the generation of artificial supercooling (SSF) was attributed to rapid freezing compared to the same freezing method without supercooling (SAF). The calculated freezing rate of pork was on the order of EIF (8.33 cm/h), SSF (0.61 cm/h) and SAF (0.37 cm/h). According to the classification of Dinçer (1997), fast freezing was defined by the freezing rate of 0.54-3.60 cm/h, hence EIF, SSF, and SAF were classified by ultra-fast, fast and slow freezing, respectively.
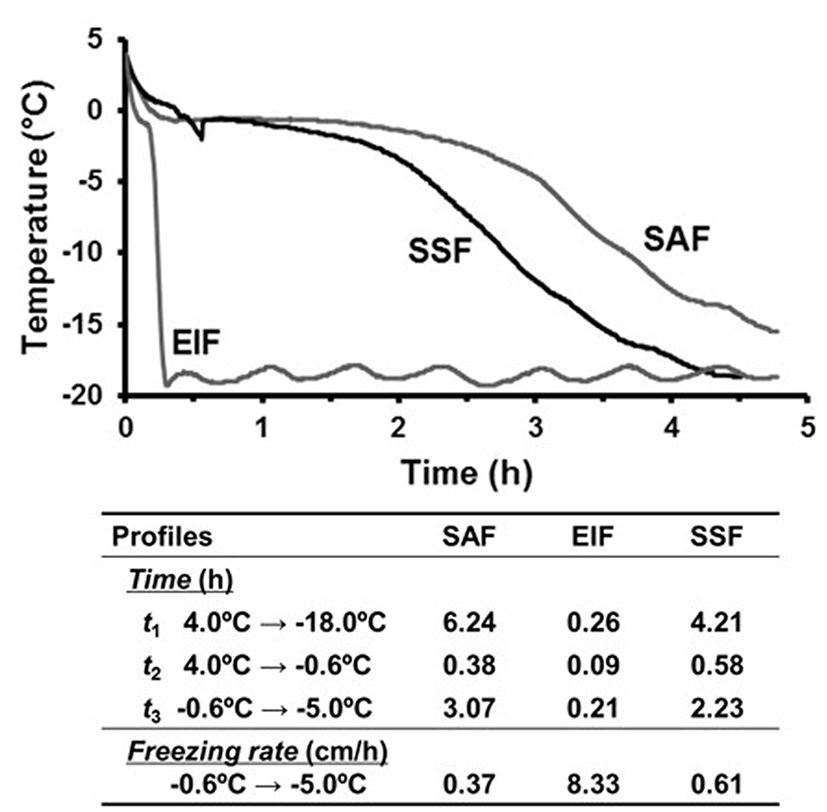
Due to difference in surface heat transfer coefficients, liquid immersion type freezing (EIF) was more rapid than air convection type (SAF and SSF) (Devine et al., 1996). For air convection type freezing, the more rapid freezing rate of SSF than that of SAF could be explained by the artificial supercooling generating a greater number of ice crystals, which would facilitate a release of latent heat (Luscher et al., 2005). Consequently, the result indicated that the extent of supercooling affected the freezing rate of pork, and the artificial supercooling had a potential advantage in reducing overall freezing time of pork.
Evidence of ice crystals generated in the frozen pork was observed from each lyophilized meat sample (Fig. 2). Like the report from Ngapo et al. (1999a), the sizes of ice crystals depended on the freezing rate. Formations of large ice crystals were evidenced in SAF treatments in which the pork loin lost its structural integrity regardless of the direction of muscle fiber. On the other hand, the microstructure of pork was rarely affected by ultra-fast freezing treatment (EIF), showing small and uniform cavities in the cross section of the sample. In the longitudinal section, no damage of EIF treatment was obtained, and the result again confirmed the importance of rapid freezing to minimize tissue damage of meat.
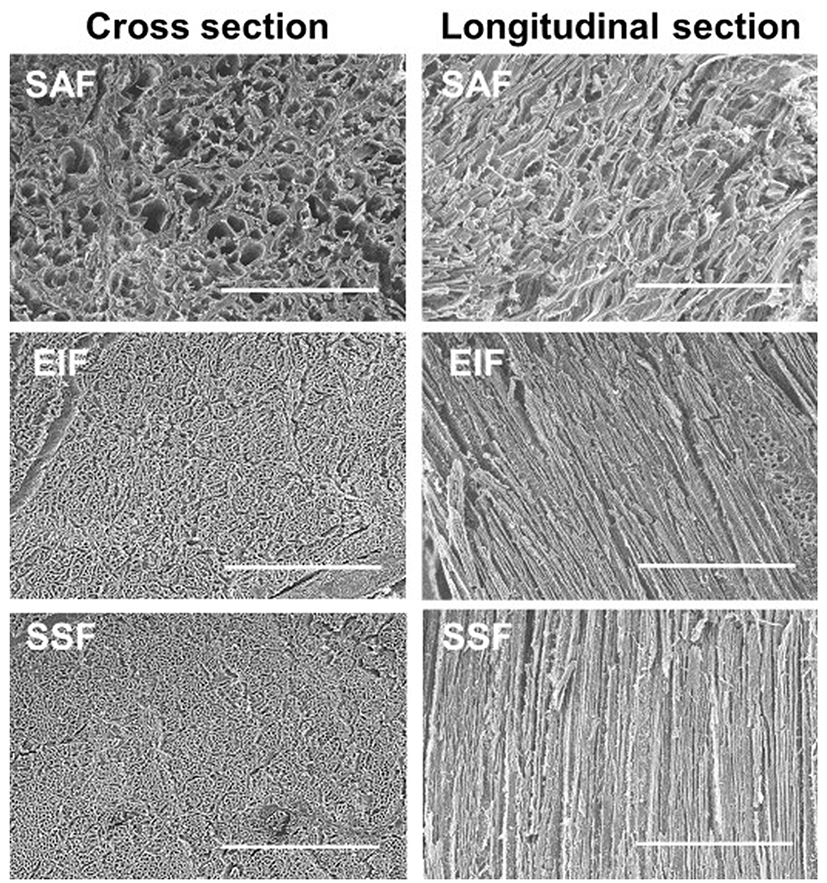
It was intriguing that the microstructure of SSF was similar to that of EIF. Despite the fact that SSF and SAF adopted same freezing method (still air freezing), the microstructure of SSF indicated that meat was hardly damaged by the slow freezing if artificial supercooling was applied. As hypothesized, supercooling triggered the formation of numerous ice nuclei, and the nucleation resulted in releasing greater latent heat. Subsequently, the rapid removal of heat inside the meat suppressed the growth of ice crystals, thereby minimizing the tissue damage of meat (Burke et al., 1976).
Based on the results, SSF exhibits greater advantage than EIF for meat freezing technique, since commercial frozen meat is not produced by liquid immersion type, but rather air convection system is normally used. Although, the impact of extent variations of supercooling on the number and size of ice crystals in pork was not completely understood in the present study, acceleration of the freezing rate without tissue damage in pork was possible by providing artificial supercooling.
The estimated water-holding capacity of frozen pork is given in Table 1. SAF treatment exhibited 6.73% of the highest thawing loss among the treatments (p<0.05). Because SAF was classified by the slowest freezing among all treatments, the result was in accordance with previous investigations (Ngapo et al., 1999b). The thawing loss of EIF and SSF treatments ranged from 4.44-4.49%, which was slightly higher than the 3.35% of the control, although the difference was not significantly different. Considering the limited sample size (2 cm in shortest direction), the difference in thawing loss reflected that the partial tissue damage in pork during freezing was unavoidable (Petrović et al., 1993). Nevertheless, the tissue damage was minimized by applying immersion-type freezing (EIF) or artificial supercooling followed by normal freezing (SSF), which was supported by the observation of microstructure.
1)CT, control; SAF, still air freezing; EIF, ethanol immersion freezing; SSF, artificial supercooling followed by still air freezing.
2)Estimated by drip loss of fresh meat after 24 h of chilled storage.
a-cMeans with difference superscript within same column are significantly different (p<0.05).
The cooking loss and expressible moisture of treatments was also showed a similar pattern to thawing loss. Among the treatments, SAF had a significantly higher cooking loss and expressible moisture than those of control (p<0.05). The cooking loss and expressible moisture of EIF and SSF were also slightly higher than those of the control, whereas the difference was not significant. Since cooking loss and expressible moisture were closely related with juiciness of meat (Devine et al., 1996; Mallikarjunan, 2006), it was expected that EIF and SSF would have a higher sensory score than SAF.
There is no doubt that the degree of tissue damage is crucial to predict quality of frozen meat (Añón and Calvelo, 1980). The physical damage in meat structure was reflected by a loss of moisture during thawing, hence a previous study postulated that meat temperature should be lowered as quickly as possible during phase transition process (Devine et al., 1996). In this case, the application of artificial supercooling had a potential benefit of accelerating the freezing rate and thereby suppressing the growth of ice crystals. Consequently, SSF could prevent moisture loss of pork even if conventional slow freezing was adopted.
As depicted in Fig. 3, the unfrozen control showed 53.8 N of shear force, and the shear force of all frozen samples was significantly higher than that of the control (p<0.05). Among treatments, SAF had 71.1 N of the highest shear force (p<0.05), while those of EIF and SSF were not significantly different, ranging between 64.6-66.5 N. It was reported that the shear force of meat had a positive correlation with cooking loss (Barbanti and Pasquini, 2005). It was believed that a denaturation of myofibrillar protein in the frozen sample was manifested by moisture loss of sarcomere as a form of thawing drip (Petrović et al., 1993). The protein denaturation affected a larger amount of cooking loss, thereby resulting in loss of tenderness. Because the protein denaturation intensely occurred by slow freezing, the highest shear force of SAF was in agreement with the finding from Petrović et al. (1993). Meanwhile, the authors also suggested that the best tenderness was found at the freezing rate of 3.95 cm/h, and the meat was less tender when the freezing rate was higher (5.55 cm/h), which was not consistent with this study. This could be explained by freezing condition, and Petrović et al. (1993) tried to freeze meat at -78℃ to obtain a 5.55 cm/h freezing rate. In this condition, the final meat temperature was lower than its eutectic point (-62℃) where the saturated solution was solidified, and meat protein underwent severe denaturation (Petrović et al., 1993; Tarrant, 1982). Meanwhile, the freezing temperature of EIF and SSF in this study did not exceed below the eutectic point and the relatively high freezing rate of these treatments enabled to possess lower shear force than that of SAF.
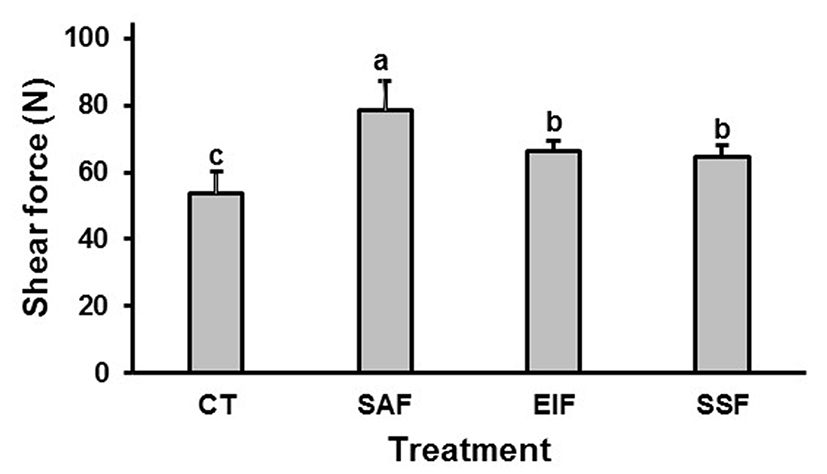
Freezing did not affect the color of pork, irrespective of freezing method (Table 2). All frozen treatments tended to show slightly lower L* and b* with higher a* than those of the control. However, the color values were not significantly different among the samples. This was probably due to short storage periods (24 h) of all treatments (Leygonie et al., 2012).
Treatment1) | L* | a* | b* |
---|---|---|---|
CT | 48.8±1.05 | 11.8±1.33 | 5.82±2.10 |
SAF | 45.6±3.92 | 12.4±0.44 | 5.10±1.14 |
EIF | 44.2±2.29 | 14.9±1.09 | 6.06±1.61 |
SSF | 47.6±3.70 | 11.1±1.71 | 4.90±1.48 |
1)CT, control; SAF, still air freezing; EIF, ethanol immersion freezing; SSF, artificial supercooling followed by still air freezing.
Conclusion
This study demonstrated that SSF treatment showed advantages in accelerating the freezing rate with minimal quality deterioration normally found in slowly frozen meat. For the main freezing process (secondary freezing stage), SSF adopted still air freezing (slow freezing), nevertheless, the impact of SSF on the meat qualities was as high as that obtained from very fast freezing (immersion freezing). Still, the impact of the extent of supercooling on the quality of meat was not fully understood in the current study, which warrants further exploration.