Introduction
Meat is a major animal-derived protein resource, containing all essential amino acids for human nutrition (Li et al., 2022b; Zheng et al., 2022a). However, conventional meat production processes such as livestock farming and feed production release significant amounts of greenhouse gases, consume vast quantities of land and water resources, and contribute to soil erosion and water pollution (Bomkamp et al., 2022; Chen et al., 2023a). Recently, the demand for meat has increased owing to urbanization and economic development (Lu et al., 2022). In addition, animal welfare issues such as large-scale intensive livestock farming have raised concerns regarding the sustainability of meat production techniques (Bomkamp et al., 2022). For example, agriculture utilizes 92% of global freshwater resources yearly, with 29% used for animal farming (Gerbens-Leenes et al., 2013). In addition, annual greenhouse gas emissions from meat production range from 4.6 to 7.1 billion tons, constituting 15%–24% of global emissions (Fiala, 2008). Therefore, the development of transformative technologies for sustainable meat production is imperative.
Cellular agriculture, which involves the production of agricultural products using cell culture techniques, is receiving attention as an innovative technology because of its potential to address food security and sustainability (Wang et al., 2024e). Cultured meat, a major product of cellular agriculture, was reported to decrease environmental impacts regarding land and water use, compared to conventional meat (Bhat et al., 2015; Djisalov et al., 2021; Tuomisto and Teixeira de Mattos, 2011). Cultured meat offers ethical advantages by minimizing animal use while producing large quantities of muscle tissue. It also contributes to environmental sustainability by reducing greenhouse gas emissions, particularly nitrous oxide released from animal waste (Chriki et al., 2022; Djisalov et al., 2021). Additionally, the risk of Salmonella and Listeria associated with conventional meat can be avoided without the use of antibiotics (Post et al., 2020).
The production of cultured meat through cellular agriculture technology is based on four fundamental components: cells, cultured media, scaffolds, and biological processes (Murugan et al., 2024; Santos et al., 2023). Scaffolds play an important role in imitating the three-dimensional (3D) structure of conventional meat. Scaffolds can be fabricated from various biomaterials through techniques such as freeze-drying, 3D bioprinting, electrospinning, and electrospray, supporting an integrated network for cell attachment, proliferation, and differentiation (Bezjak et al., 2024; Kumar et al., 2023; Levi et al., 2022; Wei et al., 2023). The structural network of scaffolds depends on the selected biomaterials and fabrication techniques, and thus affects the physicochemical and mechanical properties of cultured meat (Kumar et al., 2023; Levi et al., 2022; Santos et al., 2024). In addition, as scaffolds are directly ingested with cultured meat, it is crucial to ensure both their nutritional value and safety for human consumption (Guo et al., 2024; Wang et al., 2024b).
Recently, various studies have been conducted to evaluate the physicochemical and biological properties of scaffolds using edible biomaterials for cultured meat production (Lee et al., 2024a; Lee et al., 2024b; Wang et al., 2024a; Wang et al., 2024c). The choice of scaffold materials should be guided by the need to closely replicate the optimal properties of the target tissue, while also providing the necessary support for cell adhesion, proliferation, and differentiation (Samandari et al., 2023; Xiang et al., 2022). In fact, although scaffold biomaterials have been predominantly discussed in the field of tissue engineering, there is still a lack of knowledge regarding the optimal scaffold biomaterials for producing cultured meat. Therefore, the primary objective of this review was to provide a comprehensive understanding of the biomaterials used in cultured meat scaffolds, with the goal of informing the selection of optimal materials for efficient cultured meat production.
Animal-Derived Biomaterials
Animal-derived biomaterials offer great potential as scaffold sources due to their edible and biodegradable properties (Wang et al., 2023a). These are primarily categorized into polysaccharides (chitosan), proteins (collagen, gelatin, and fibrin), and polynucleotides (Seah et al., 2022). These biomaterials provide a native environment that support cell growth and differentiation into tissues in cultured meat (Reiss et al., 2021). In particular, the components of the extracellular matrix (ECM) are ideal biomaterials because of their similarity to the texture and structure of conventional meat (Tarafdar et al., 2021). However, quality control challenges, such as ensuring safety against pathogens and maintaining consistent properties, along with high production costs, prevent the widespread use of animal-derived biomaterials. Importantly, the excessive use of animal ingredients may compromise the fundamental objectives of cultured meat production, like animal welfare (Ahmad et al., 2021; Lee et al., 2024a). Table 1 summarizes the characteristics of animal-derived biomaterials. Chitosan offers biocompatibility and biodegradability but requires additional biomaterials to improve structural integrity. Collagen provides cell adhesion sites and biocompatibility but lacks mechanical strength. Gelatin supports cell adhesion and growth but has limitations in gel formation owing to its low melting point. Fibrin offers biocompatibility but is limited by its high cost. Therefore, animal-derived biomaterials should be combined with other materials to achieve optimal scaffold properties for cultured meat.
Type | Biomaterial | Strength | Limitation | Reference |
---|---|---|---|---|
Polysaccharide | Chitosan | Food-grade biomaterials, contain cross-linking functional groups, resemble glycosaminoglycans, provide a microenvironment for cell adhesion and proliferation | Weak mechanical properties, low structure integrity | Chen et al. (2010); Cooper et al. (2010); Li et al. (2022b); Ul-Islam et al. (2024); Wu et al. (2024); Zernov et al. (2022) |
Protein | Collagen | Contain Arg-Gly-Asp (RGD) motif, repetitive receptor-recognition motifs, promote cell adhesion and cell interaction, high biocompatibility, low immunogenicity, biodegradability, edible | High cost, low mechanical strength | Chen et al. (2024a); Davidenko et al. (2015); Li et al. (2022b); Wang et al. (2024d); Zernov et al. (2022) |
Gelatin | Contain RGD sequences, support cell adhesion and growth, safety, biocompatible, biodegradable, mechanical support, contain intrinsic integrin-binding domains | Low melting point, low shape stability, poor mechanical strength, low elasticity | Chen et al. (2023b); Kong et al. (2022); Li et al. (2022a); Rao et al. (2023); Xing et al. (2014) |
|
Fibrin | Contain As, Bβ, and γ peptide chains, biocompatibility, bind proteins and growth factors | High production cost, weak mechanical properties | Contessi Negrini et al. (2020); Haugh et al. (2012); Rojas-Murillo et al. (2022); Tan et al. (2021) |
Chitosan, an abundant natural polymeric polysaccharide, is typically derived from waste products of the shellfish industry and can also be produced from non-animal sources, such as fungi (Wang et al., 2024d; Xie et al., 2024). Chitosan is a food-grade biomaterial that is generally recognized as safe (GRAS; Zernov et al., 2022). Chitosan contains abundant amine (-NH2) and hydroxyl (-OH) groups able to crosslink with cells and share structural similarities with glycosaminoglycans, a component of the ECM (Li et al., 2022b; Wu et al., 2024). Accordingly, the structure of chitosan provides a microenvironment for cell adhesion and proliferation, contributing to desirable properties for scaffold fabrication (Chen et al., 2010; Cooper et al., 2010). However, chitosan must be combined with other biomaterials to improve its structural integrity because of its weak mechanical properties when used as a standalone material (Ul-Islam et al., 2024). A previous study incorporated 2% chitosan with 2% sodium alginate, 0.5% collagen, and 0.5% gelatin (2:2:1:1 ratio) through electrostatic interactions to improve mechanical properties of the scaffold (Li et al., 2022b). This scaffold exhibited laminar porous structures with interconnected fibrils between the layers (Li et al., 2022b). This enhanced the compressive strength and promoted the proliferation and differentiation of porcine skeletal muscle satellite cells. In addition, microcarriers supplemented with 2% chitosan and 1% collagen (9:1 ratio) significantly enhanced viability and proliferation of primary rabbit smooth muscle cells, sheep fibroblasts, and bovine umbilical cord mesenchymal stem cells (Zernov et al., 2022). Collectively, chitosan can be employed in scaffold fabrication to enhance mechanical strength and biocompatibility when combined with other biomaterials.
Collagen is the primary component of the muscle connective tissue and is extracted from the ECM (Zheng et al., 2022a). Collagen contains abundant Arg-Gly-Asp (RGD) motifs and repetitive receptor-recognition motifs that promote cell adhesion and cell interaction (Chen et al., 2024a; Davidenko et al., 2015; Zernov et al., 2022). Furthermore, collagen has inherent characteristics, such as high biocompatibility, low immunogenicity, and biodegradability, which make it suitable for use as an edible scaffold material (Li et al., 2022b; Wang et al., 2024d). However, the high cost and low mechanical strength of collagen remain challenges for its application as a biomaterial for scaffolds (Li et al., 2022b; Zernov et al., 2022). To address these limitations, a previous study developed a scaffold fabricated with 1% collagen, 5% proanthocyanidins, and 5% dialdehyde chitosan in a ratio of 88:4:8 through electrostatic interactions and Schiff base reactions (Wang et al., 2024d). The collagen-proanthocyanidins-dialdehyde chitosan scaffold exhibited superior mechanical (microstructure and compression strength), physical (porosity, swelling ratio, and degradation ratio), and biological (adhesion, proliferation, differentiation of primary skeletal muscle myoblasts) properties, compared to pure collagen scaffold (Wang et al., 2024d). In addition, a prior study fabricated an aligned porous scaffold crosslinked with 4% collagen and 30 U/g transglutaminase through ice-templated directional freeze-drying to improve the cost and structure of cultured meat (Chen et al., 2024a). The cultivation of porcine skeletal muscle satellite cells on this aligned porous scaffold improved mechanical strength (microstructure, hardness, chewiness, and resilience), proliferation (live cell fluorescence), and differentiation (F-actin fluorescence, myogenin, and myosin; Chen et al., 2024a). In scaffold fabrication, collagen can become a suitable biomaterial through the establishment of methods to enhance mechanical strength and reduce costs in combination with various biomaterials.
Gelatin is a product of collagen hydrolysis containing RGD sequences that support cell adhesion and growth (Chen et al., 2023b; Rao et al., 2023). The U.S. Food and Drug Administration (FDA) considers gelatin to be safe, biocompatible, and biodegradable (Li et al., 2022a; Rao et al., 2023). Gelatin has been employed as a mechanical support for cell attachment due to its intrinsic integrin-binding domains (Kong et al., 2022). However, since gelatin has a relatively low melting point of approximately 28°C–30°C, a gelatin hydrogel formed through non-covalent associations below 30°C–35°C is easily destroyed at physiological temperatures of 37°C (Xing et al., 2014). Therefore, the gelatin hydrogel possesses low shape stability, poor mechanical strength, and low elasticity, limiting its application in cultured meat production. These limitations can be addressed by inducing covalent crosslinking to enhance mechanical stability and by employing as a coating material to improve biocompatibility. According to a previous study, various concentrations of gelatin (0%, 0.5%, and 1%) were coated to scaffold fabricated with 5% soy protein and 2% agarose in a 1:1 ratio (Hong et al., 2024). The 1% gelatin-coated scaffold increased water absorption rate, mechanical strength, cell attachment, and lipid accumulation in adipose tissue-derived stem cells compared to the non-coated scaffold (Hong et al., 2024). Additionally, textured vegetable protein was coated with 6% gelatin and agar at ratios of 4:0, 4:0.5, 4:1, and 4:3 (Lee et al., 2022). The gelatin and agar coating at a ratio of 4:1 demonstrated optimal hydrogel stiffness and stability comparable to muscle and enhancing cell attachment, proliferation, and mechanical strength (Lee et al., 2022). Considering these previous studies, the enhancement of mechanical properties in gelatin enables its application as a biomaterial in cultured meat production.
Fibrin is a byproduct of fibrinogen, which is composed of As, Bβ, and γ peptide chains (Tan et al., 2021). Fibrin is a suitable biomaterial for scaffolds owing to its biocompatibility and ability to bind proteins and growth factors (Rojas-Murillo et al., 2022). However, fibrin produced from human thrombin and fibrinogen has a high production cost (Contessi Negrini et al., 2020). Furthermore, the weak mechanical properties of fibrin hydrogel due to its hydrated nature (i.e. the low protein to water ratio) limits its use in cultured meat application (Haugh et al., 2012). To overcome these limitations, a study developed hydrogel scaffolds combined with fibrin and konjac glucomannan at a ratio of 3:2 (Tang et al., 2024). The 1.2% fibrin and 0.8% konjac glucomannan hydrogels induced glycosylation through hydrogen-bond interactions and possesed suitable degradation rate, water holding capacity, textural properties, and biocompatibility for cultured meat production (Tang et al., 2024). Moreover, a fibrin hydrogel mixed with 10 mg/mL fibrinogen and 5 U/mL thrombin was fabricated in a 15 mm long section of silicone tube tissue mold, cultivating piscine satellite cells at a concentration of 6.0×106 cells/mL for cultured fish meat production (Lou et al., 2024). This fibrin matrix exhibited superior biocompatibility (cell viability, proliferation, differentiation, and alignment) along with textural and nutritional similarity to natural fish fillets (Lou et al., 2024). These reports indicate that fibrin, while promising for cultured meat when combined with other biomaterials or structurally aligned, requires cost-effective separation and extraction methods for widespread adoption.
Plant-Derived Biomaterials
Plant-derived biomaterials are an attractive option for scaffold fabrication in cultured meat, given that the primary purpose of cultured meat is to reduce the reliance on animal-derived materials (Kim et al., 2024). Also, the fibrous structure of plant-derived biomaterials closely resembles that of conventional meat, making them well-suited for use as scaffolds (Zheng et al., 2022b). Plant-derived biomaterials are classified into polysaccharides and proteins, offering technical and economic advantages such as high nutritional value, biocompatibility, consumer acceptance, and low cost (Ben-Arye and Levenberg, 2019; Ng and Kurisawa, 2021). However, the limited cell attachment of these biomaterials has driven recent research efforts towards developing plant-based scaffolds through the utilization of various scaffolding technologies (Levi et al., 2022; Rao et al., 2023). Table 2 presents the properties of the plant-derived biomaterials in the scaffolds. Polysaccharides such as cellulose, starch, and glucomannan offer biocompatibility, biodegradability, and low cost, making them suitable for cultured meat scaffolds. Proteins, including soy protein, pea protein, zein, and glutenin, enhance cell adhesion, proliferation, and differentiation and have high nutritional value. Decellularized plant-derived materials provide structural support and vascular systems as scaffolds for cultured meat.
Type | Biomaterial | Strength | Limitation | Reference |
---|---|---|---|---|
Polysaccharide | Cellulose | Biocompatibility, non-toxic, eco-friendliness, supports cell proliferation and differentiation | Non-specific protein adsorption, limiting cell adhesion | Courtenay et al. (2017); Klemm et al. (2005); Siró and Plackett (2010) |
Starch | Biodegradability, high availability, low cost, non-toxic | Low mechanical strength, high hydrophilicity | Apriyanto et al. (2022); Buléon et al. (1998); Torres et al. (2013) |
|
Glucomannan | Excellent gelling and water-holding properties | Lack of hydrophobicity and viscosity, low thermal stability and mechanical strength | Ran and Yang (2022); Ran et al. (2022); Ye et al. (2021); Zhuang et al. (2024) |
|
Protein | Soy protein | High nutritional value, food safety, low cost | Low mechanical properties, insufficient water-resistance | Chien and Shah (2012); Milani and Tirgarian (2020); Mohammadian and Madadlou (2018); Sui et al. (2021); Tian et al. (2018) |
Pea protein | High nutritional content, low allergenicity, availability, affordability, low cost | Low solubility, high denaturation temperature | Başyiğit et al. (2024); Estevinho and Rocha (2018); Li et al. (2020); Shanthakumar et al. (2022); Stone et al. (2015) |
|
Zein | Biocompatibility, biodegradability, amphiphilicity, self-assembly | Hydrophobicity and deficiency of essential amino acids | Falsafi et al. (2023); Giteru et al. (2021); Wang et al. (2022); Zhang et al. (2023) |
|
Glutenin | High nutritional value, low cost, biocompatibility | Limited processability, low solubility | Xu et al. (2014); Yao et al. (2024) |
|
Decellularized plant-derived materials | Parsley | Supports cell proliferation and differentiation, provides a vascular system for supplying oxygen and nutrients, low cost, edibility | Different structural and functional properties dependent on the type of plant | Chen et al. (2024b); Contessi Negrini et al. (2020); Jones et al. (2023); Murugan et al. (2024); Thyden et al. (2022) |
Apple | ||||
Banana leaf | ||||
Spinach | ||||
Celery | ||||
Mushroom |
Cellulose, a natural polymer found in plant cell walls, is composed of D-glucose units linked by β-1,4 glycosidic bonds (He et al., 2021). Cellulose has been widely used in the food and pharmaceutical industries because of its biocompatibility, non-toxicity, and eco-friendliness (Klemm et al., 2005; Siró and Plackett, 2010). However, the hydrophilicity of natural cellulose due to its hydroxyl groups reduces non-specific protein adsorption, thereby limiting cell adhesion (Courtenay et al., 2017). To overcome these limitations, cellulose derivatives are frequently employed in scaffold fabrication. These derivatives, including cellulose acetate and carboxymethylcellulose, are modified by substituting the hydroxyl groups of natural cellulose with acetyl and carboxyl groups (Park et al., 2021; Santos et al., 2024). Cellulose nanofiber scaffolds in random or aligned forms have been fabricated using a 12% cellulose acetate solution via electrospinning. The random cellulose acetate nanofibers with a porous structure have supported suitable adhesion and differentiation of C2C12 and H9c2 myoblasts, demonstrating their potential application for cultured chicken meat (Santos et al., 2024). In addition, a polysaccharide film platform has been developed to produce cost-effective cultured meat via the replacement of animal-derived serum with C-phycocyanin extracted from blue algae. The polysaccharide films were fabricated into multilayer structure composed of carboxymethylcellulose and chitosan through electrostatic interaction-based layer-by-layer assembly process. These film platforms provided a porous structure for effective incorporation and release of C-phycocyanin, promoted the proliferation of C2C12 cells, and achieved a 4-fold improvement (Park et al., 2021). Collectively, cellulose derivatives, synthesized by modifying natural cellulose through hydroxyl group substitution, can serve as scaffold biomaterials to enhance cell adhesion and proliferation.
Starch is a biodegradable carbohydrate polymer that is mainly found in corn, wheat, tapioca, rice, and potatoes (Buléon et al., 1998). Owing to its high availability, low cost, nontoxicity, and biodegradability, starch has been widely applied in various industries, including the food industry (Apriyanto et al., 2022). Since the low mechanical strength and high hydrophilicity of starch limit its application as a biomaterial for scaffold fabrication, various studies have been conducted to address these challenges (Torres et al., 2013). Indeed, starch-based scaffolds were developed using 3D printing techniques with 5 g of starch gel combined with 0–0.1 g of calcium carbonate and glucono delta lactone in a 1:2 ratio (Wang et al., 2024a). The addition of calcium carbonate and glucono delta lactone improved mechanical (compression modulus), structural (microstructure, pore size), physical (swelling ratio, digestibility, and water stability), and biological (proliferation and differentiation of C2C12 myoblasts) properties of starch-based scaffolds (Wang et al., 2024a). Additionally, a previous study developed a 3D bioprinting bioink by incorporating 1% and 5% starch nanoparticles into hydrogels composed of 15% gelatin and 1% sodium alginate (Niu et al., 2024). The addition of 1% starch nanoparticles to the gelatin-based hydrogels reduced the viscosity and shear stress of the bioink and increased the proliferation and differentiation of piscine satellite cells, thereby enhancing 3D printability and biocompatibility (Niu et al., 2024). Collectively, starch can be efficiently utilized through either combination with other biomaterials or structural modification into nanoparticles (Lu and Tian, 2021; Niu et al., 2024).
Glucomannan is a polysaccharide in the mannan family that is commonly found in softwood, roots, tubers, and plant bulbs (Alonso-Sande et al., 2009). Among the various types of glucomannans, the most utilized form is konjac glucomannan, which is extracted from Amorphophallus konjac tubers (Xiao et al., 2000a; Xiao et al., 2000b). Konjac glucomannan has been extensively utilized in food and tissue engineering industries owing to its gelling and water holding properties (Ran and Yang, 2022; Ran et al., 2022; Ye et al., 2021). Nevertheless, pure konjac glucomannan exhibits limitations, including low hydrophobicity, viscosity, thermal stability, and mechanical strength (Zhuang et al., 2024). To mitigate these limitations, previous studies have investigated the fabrication of hydrogels by constructing composites of konjac glucomannan with biomaterials such as fibrin or k-carrageenan (Gu et al., 2024; Tang et al., 2024). Konjac glucomannan-based hydrogel composites exhibited enhanced mechanical (hardness and chewiness), physical (viscoelasticity, degradability, and water holding capacity), and biological (cell proliferation and differentiation) properties (Gu et al., 2024; Tang et al., 2024). Therefore, the incorporation of konjac glucomannan with other biomaterials can be an efficient strategy for scaffold fabrication in cultured meat production.
Soy protein is a popular alternative to animal-derived proteins because of its high nutritional value, reliable food safety, and low cost (Chien and Shah, 2012; Mohammadian and Madadlou, 2018; Sui et al., 2021). However, low mechanical properties and insufficient water resistance must be addressed to effectively utilize soy protein as a scaffold material (Milani and Tirgarian, 2020; Tian et al., 2018). For this, a previous study developed aligned porous scaffolds incorporated with 5% soy protein amyloid fibrils and glycerin at ratios of 70:30, 60:40, 50:50, and 40:60 through the unidirectional freeze casting method (Shan et al., 2024). The increase of glycerin content in soy protein-based scaffolds resulted in the formation of smaller and more regular pores, and this reduction in porosity enhanced water resistance and mechanical strength (Shan et al., 2024). In another study, scaffolds composed of 5% soybean protein isolate, 0.5% soybean dietary fiber, and 5% glycerol were crosslinked with both 5.5% transglutaminase and 5.8% calcium chloride (Fang et al., 2024). These scaffolds increased mechanical strength (compression modulus), water resistance (degradation ratio), and biocompatibility (cell viability) compared to those fabricated using transglutaminase or calcium chloride. It seems that the enhancement of water resistance and mechanical strength through the incorporation of other biomaterials is essential for utilizing soy protein as a scaffold biomaterial.
Pea protein is an attractive biomaterial characterized by its high nutritional content, low allergenicity, availability, affordability, and low cost (Li et al., 2020; Stone et al., 2015). Due to these functional properties, pea protein is extensively utilized as a promising ingredient in the food industry (Shanthakumar et al., 2022). Moreover, recent studies have expanded the application of pea protein as a biomaterial for scaffold fabrication in cultured meat production (David et al., 2024; Ianovici et al., 2022). However, the low solubility and high denaturation temperature of pea protein due to a broad range of the isoelectric point from pH 4 to 6 limit its gelling properties for scaffold fabrication (Başyiğit et al., 2024; Estevinho and Rocha, 2018). To address this limitation, a previous study fabricated mold-based scaffolds incorporating 15% pea protein isolate at pH 2 and 7 (David et al., 2024). Pea protein isolates at both pH 2 and 7, combined with 2% alginate, effectively induced gelation in polydimethylsiloxane molds through crosslinking with calcium chloride. Although there were no differences in the physical properties (porosity, connectivity, morphology, and liquid absorption) of scaffolds between pH 2 and 7, the scaffolds at pH 7 exhibited higher proliferation and differentiation of bovine satellite cells compared to those at pH 2 (David et al., 2024). Additionally, 1% pea protein isolates and 1% alginate were mixed in 1:1 ratio to fabricate mold-based scaffold (Ianovici et al., 2022). Pea protein isolates-alginate scaffolds enhanced the mechanical (Young’s modulus), physical (porosity, degradability, and liquid uptake), and biological (proliferation and differentiation of bovine satellite cells) properties compared to single alginate scaffolds (Ianovici et al., 2022). Taken together, despite its limitations in solubility and denaturation, pea protein can be utilized for cultured meat scaffold fabrication through optimization of pH and incorporation with alginate, resulting in scaffolds that support cell proliferation and differentiation.
Zein, as a GRAS, is a prolamin protein produced from corn grains via a wet milling process (Falsafi et al., 2023). The biocompatibility, biodegradability, amphiphilicity, and self-assembly of zein can be ideal biomaterials for scaffold fabrication in cultured meat production (Wang et al., 2022). The high percentage of sulfur-containing amino acids in zein leads to hydrophobicity and a deficiency in essential amino acids, thereby restricting its applications (Giteru et al., 2021; Zhang et al., 2023). A prior study injected a 1% hydrophilic sodium alginate solution into a coagulation bath containing 30% zein and 5% calcium chloride solution through a wet-spinning technique to fabricate zein-alginate fiber (Jeong et al., 2024). Zein-coated alginate fibers exhibited higher tensile stress and elastic modulus than alginate fibers. Furthermore, zein-alginate fibers subjected to a 75% strain enhanced alignment and myogenesis during the cultivation of C2C12 and bovine satellite cells (Jeong et al., 2024). In another study, zein short-stranded fibers, generated from 28% zein solutions by means of electrospinning and ultrasonication, were integrated into RGD-functionalized alginate hydrogels (Melzener et al., 2023). The addition of 0.1% zein fibers into 1.8% alginate hydrogels improved biomaterial degradation, cellular compaction, metabolic activity, and protein productivity (Melzener et al., 2023). Considering these characteristics of zein in scaffold fabrication, hydrophobic zein can be effectively utilized when incorporated with hydrophilic biomaterials.
Glutenin, which consists of gliadin and glutenin, is one of the two main components of wheat gluten. Glutenin is composed of aggregated proteins characterized by interchain disulfide bonds (Abedi and Pourmohammadi, 2021; Wieser, 2007). Glutenin is regarded as a promising biomaterial for scaffold fabrication due to its high nutritional value, cost-effectiveness, and biocompatibility (Yao et al., 2024). Despite these advantages, the highly crosslinked molecular structure of pure glutenin significantly restricts its processability and solubility (Xu et al., 2014). To address this issue, a previous study engineered porous and fibrous glutenin scaffolds. This was achieved by acidifying a 5% glutenin solution to pH 3 and subsequently modifying its secondary structure via water annealing (Xiang et al., 2022). These scaffolds effectively supported the proliferation, differentiation, and myogenesis of C2C12 and bovine satellite cells (Xiang et al., 2022). In another study, the combination of 3% glutenin of pH 3 dissolved in water with 1.5% chitosan at ratio of 1:1 enabled the formation of 3D porous scaffold through water annealing (Wu et al., 2024). The addition of chitosan into glutenin-based scaffolds enhanced structural (pore size, porosity, and swelling), physical (thermal stability, disulfide bonds, secondary structure), and biological (proliferation and differentiation of porcine satellite cells) properties through the increase of -NH2 and -OH groups (Wu et al., 2024). Consequently, increasing glutenin solubility through pH adjustment or by combining it with other biomaterials can improve processability, thereby enabling the fabrication of scaffolds.
Decellularization is the process of removing all cellular components, leaving the tissue structure and ECM (Contessi Negrini et al., 2020; Murugan et al., 2024; Thyden et al., 2022). Decellularized plant sources are ideal biomaterials for scaffolds because of their structural and functional properties that support cell adhesion, proliferation, and differentiation through the vascular system of oxygen and nutrients (Chen et al., 2024b; Jones et al., 2023). Furthermore, the edibility and low cost of decellularized plant-derived materials contribute to the production of safe and economical scaffolds for cultured meat. However, the structural and functional properties of decellularized plant scaffolds exhibit significant variability depending on the plant source (Chen et al., 2024b). Previous studies have explored the potential of various decellularized plant materials, including parsley, spinach, and banana leaves, as scaffold sources. Decellularized parsley scaffolds promote the proliferation and differentiation of C2C12 myoblasts by forming longitudinal and transverse pore structures (Chen et al., 2024b). Similarly, primary bovine satellite cells cultured on decellularized spinach leaves maintain 99% viability and 25% differentiation compared to gelatin-coated glass slides (Jones et al., 2021). Decellularized celery scaffolds support chicken myoblast proliferation and differentiation, with fully grown myoblasts completely covering the scaffold surface and forming fiber-like myotube structures (Hong and Do, 2024). Additionally, various plant-derived sources, including banana leaves, mushrooms, and apples, have been decellularized as scaffolds for cultured meat production (Banavar et al., 2024; Singh et al., 2023; Sood et al., 2024). Therefore, considering biocompatibility, safety, and economic efficiency, the decellularization of plant-derived materials can be an effective biomaterial for cultured meat scaffolds.
Algae-Derived Biomaterials
Algae are a diverse group of photosynthetic organisms that inhabit aquatic environments and have been consumed by humans for centuries (Wells et al., 2017). Algae are considered safe food products that contain abundant bioactive compounds, such as polysaccharides, proteins, bioactive peptides, fatty acids, and vitamins (Diaz et al., 2022). Algae are generally categorized into macroalgae and microalgae based on their size (Wang et al., 2023b). Macroalgae are multicellular organisms classified into three groups: red seaweed (Rhodophyceae), brown seaweed (Phaeophyceae), and green seaweed (Chlorophyceae). In contrast, microalgae are unicellular organisms, consisting of diatoms (Bacillariophyceae), green algae (Chlorophyceae), golden algae (Chrysophyceae), and blue-green algae (Cyanophyceae; Catarina Guedes et al., 2011; Pina-Pérez et al., 2017). Microalgae are rich in proteins, such as essential amino acids and bioactive peptides, whereas macroalgae are primarily composed of polysaccharides (Afonso et al., 2019). Studies have shown that microalgae can be challenging to use as food ingredients in cultured meat scaffolds due to their pigmentation, aquatic odor, and high moisture content, which can negatively impact the texture and taste of the final product (Caporgno et al., 2020; Zhang et al., 2022). Consequently, macroalgae are often considered more suitable biomaterials than microalgae for creating edible scaffolds in cultured meat applications. Table 3 displays the characteristics of algae-derived biomaterials applicable to scaffold fabrication. While carrageenan, alginate, and agarose exhibit excellent gelling and thickening properties, their limited cell-binding sites and low mechanical stability restrict their use in scaffold fabrication. To produce cultured meat, these biomaterials often require combination with other biopolymers.
Biomaterial | Source | Strength | Limitation | Reference |
---|---|---|---|---|
Carrageenan | Red seaweed | Gel formation, resembles glycosaminoglycans, provides thickening and emulsifying properties, biocompatibility, biodegradability, non-immunogenicity, non-toxicity | High moisture content, low mechanical stability | Ãlvarez-Viñas et al. (2024); Khrunyk et al. (2020); Marques et al. (2022); Rhein-Knudsen et al. (2015); Zhang et al. (2019) |
Alginate | Brown seaweed | Gel formation through interaction with cations | Lack of cellular attachment sites | de Laia et al. (2014); Lee et al. (2024a); Wang et al. (2024c) |
Agarose | Red seaweed | Firm and porous gel formation, resembles ECM, support cell growth, high water holding capacity | Lack of cell-binding domains | Garakani et al. (2020); Hong et al. (2024a); Samrot et al. (2023); Sánchez-Salcedo et al. (2008); Zarrintaj et al. (2018) |
Carrageenan, a sulfated polysaccharide extracted from red seaweed, is composed of repeating disaccharide subunits. Carrageenan is classified into six types, ι-, κ-, λ-, θ-, ν-, and μ-carrageenan (Qamar et al., 2024). ι-Carrageenan forms a resilient gel in the presence of calcium salts. However, ι-carrageenan has a softer gel strength than κ-carrageenan due to the 2-sulfate groups on its exterior forming additional bonds through calcium interactions (Pettinelli et al., 2020). Accordingly, κ-carrageenan is mostly used in the food industry because it can form gels, similar to how natural substances called glycosaminoglycans form gels (Marques et al., 2022). At high temperatures of 75°C–80°C, κ-carrageenan exists as random coils in solution due to electrostatic repulsion between polymer chains. Upon cooling, these chains undergo a conformational change, forming aggregated helical dimers through intermolecular interactions, ultimately leading to gelation (Rhein-Knudsen et al., 2015). Therefore, κ-carrageenan serves as an effective stabilizer in food products, exhibiting thickening, gelling, and emulsifying properties (Ãlvarez-Viñas et al., 2024). κ-Carrageenan has also gained attention as a biomaterial for scaffold fabrication in cultured meat production, due to its biocompatibility, biodegradability, non-immunogenicity, and non-toxicity (Khrunyk et al., 2020). However, the inherent high moisture content of κ-carrageenan-based scaffolds contributes to substantial swelling, consequently resulting in reduced mechanical stability and increased brittleness (Zhang et al., 2019). To effectively utilize κ-carrageenan in scaffold fabrication, its mechanical strength should be improved by forming a double network structure through the incorporation of other biomaterials. A previous study developed an edible hydrogel crosslinked with κ-carrageenan and konjac glucomannan for culturing porcine adipose tissue to produce cultured meat (Gu et al., 2024). Hydrogels fabricated with single κ-carrageenan or konjac glucomannan were prone to collapse and rupture, whereas those with κ-carrageenan or konjac glucomannan ratios of 5:5, 4:6, and 3:7 exhibited superior mechanical strength (hardness and chewiness), viscoelasticity, and biocompatibility (cell viability, differentiation, and lipid content; Gu et al., 2024). In another study, Ulagesan et al. (2024) combined κ-carrageenan with sodium alginate to create a bioink for culturing fish muscle satellite cells. A mixture of 6% sodium alginate and 4% κ-carrageenan proved optimal for printing, offering enhanced antibacterial and sensory properties (Ulagesan et al., 2024). These studies suggest that κ-carrageenan holds promise as a biomaterial for improving the mechanical properties of scaffolds when combined with other biomaterials.
Alginate, an anionic polysaccharide derived from brown seaweed, is composed of β-L-guluronic acid (G-form) and α-D-mannuronic acid (M-form) in a linear polymer. Alginate can form net-structured gels through interactions with divalent cations, such as Ba2+, Ca2+, and Sr2+ (de Laia et al., 2014; Wang et al., 2024c). However, the use of pure alginate as a scaffold material is limited owing to its lack of cellular attachment sites (Lee et al., 2024a). Recent studies have focused on combining other biomaterials to overcome this limitation. An alginate-cellulose hydrogel, derived from the medulla of Undaria pinnatifida (commonly known as miyeok or sea mustard) improved structural (porosity and microstructure), physical (viscoelasticity and water holding capacity) and biological (cell adhesion, proliferation, and differentiation) properties, compared to that composed of 2% alginate alone (Lee et al., 2024a). Scaffolds composed of 2% sodium alginate and 5% gelatin at a ratio of 2:1 and coated with 0.1% tea polyphenols promoted the adhesion, proliferation, and differentiation of mouse and rabbit myoblasts (Chen et al., 2023a). Since the mechanical properties of the scaffold can be enhanced through electrostatic interactions between the carboxylate moieties of sodium alginate and the protonated amine groups of chitosan, a prior study developed 2% alginate and 2% chitosan containing scaffolds coated with 0.5% collagen and 0.5% gelatin for cultured meat production (Li et al., 2022b). These scaffolds demonstrated good structural (porosity and structural stability), physical (water holding capacity), and biological (cell viability, proliferation, and differentiation) properties (Li et al., 2022b). Interestingly, a previous study demonstrated that alginate fibers fabricated through wet-spinning with 1% sodium alginate and 11% calcium chloride exhibited a positively charged surface (Ben-Arye and Levenberg, 2019; Seo et al., 2023). This positively charged surface significantly enhanced C2C12 myoblast adhesion and viability up to 87.78% and 97.18%, respectively. Overall, these results show that alginate can be a useful material for making scaffolds for cultured meat. This can be achieved by combining alginate with other materials or by changing the way alginate is structured.
Agarose is a linear polysaccharide linked by alternating α-1,3 and β-1,4 glycosidic bonds (Samrot et al., 2023). The chemical structure of agarose is similar to that of the ECM, enabling formation of a firm and porous scaffold suitable for cell growth (Garakani et al., 2020; Zarrintaj et al., 2018). Moreover, high water holding capacity of agarose provides sufficient supplies of oxygen and nutrients for the proliferation and differentiation of cells along with its superior biocompatibility (Samrot et al., 2023; Sánchez-Salcedo et al., 2008). However, the absence of cell-binding domains in agarose significantly limits its application as a scaffold biomaterial for cultured meat (Garakani et al., 2020; Hong et al., 2024). This limitation can be addressed by structurally modifying agarose through blending with other biomaterials (Zarrintaj et al., 2018). A previous study demonstrated that crosslinking 2% agarose with 5% soy protein isolate and subsequent coating with 1% gelatin significantly enhanced mechanical strength and structural stability (Hong et al., 2024). This modification resulted in increased water absorption, mechanical strength, cell viability, and adipogenic differentiation. In 0.375% salmon gelatin and 0.375% alginate scaffolds supplemented with 0.1% glycerol, 0.25% agarose also effectively increased the growth of C2C12 myoblasts, along with improvements in microstructure and water interaction capacity (Enrione et al., 2017). Collectively, these studies suggest that the incorporation of other biomaterials to augment cell-binding domains within agarose scaffolds can significantly enhance their suitability for applications in cultured meat production.
Microbial-Derived Biomaterials
Microbial biomaterials are produced via microbial fermentation involving various genera of bacteria, yeast, and molds (Choi and Shin, 2020; Moradi et al., 2021). Polysaccharides are the most widely utilized biomaterials among six major classes: polysaccharides, polynucleotides, polyesters, polythioesters, inorganic polyanhydrides, and polyamides (Choi and Shin, 2020; Nešić et al., 2019). This widespread use of polysaccharides is primarily attributed to their desirable properties, including biodegradability, biocompatibility, and non-toxicity. Bacterial polysaccharides are categorized into cytosolic polysaccharides, lipopolysaccharides, and extracellular polysaccharides. Extracellular polysaccharides are further divided into homopolysaccharides and heteropolysaccharides depending on their monosaccharide composition (Zikmanis et al., 2020). Herein, bacterial cellulose and gellan are representative biomaterials of homopolysaccharides and heteropolysaccharides, respectively (İncili et al., 2025). Table 4 shows the properties of the microbially derived biomaterials used for scaffold fabrication in cultured meat production. Bacterial cellulose and gellan are promising biomaterials for enhancing the mechanical strength of scaffolds. However, low nutritional value of bacterial cellulose and lack of cell-binding site of gellan should be addressed for scaffold applications.
Biomaterial | Source | Strength | Limitation | Reference |
---|---|---|---|---|
Bacterial cellulose | Gluconacetobacter | Superior mechanical stability, thermostability, crystallinity, purity, surface area, permeability, porosity, water holding capacity, biodegradability | Low nutritional value due to their indigestible properties | Choi and Shin (2020); Khan et al. (2022); Tang et al. (2024); Torgbo and Sukyai (2018); Wang et al. (2024b) |
Gellan | Sphingomonas elodea | Gel formation through cation-mediated helical bonding, low water resistance | Low cell attachment due to the bioinert properties | Alharbi et al. (2024); Chen et al. (2023b); Ferris et al. (2013); İncili et al. (2025); Koivisto et al. (2019); Moxon and Smith (2016) |
Bacterial cellulose and plant cellulose share the same molecular composition, both consisting of long chains of glucose molecules. However, bacterial cellulose exhibits a unique 3D hierarchical nanofiber structure (Choi and Shin, 2020). Despite primarily being produced by Gluconacetobacter at a laboratory scale, bacterial cellulose exhibits superior properties compared to plant cellulose. These include enhanced mechanical stability, thermostability, crystallinity, and purity (free of lignin, hemicellulose, and pectin; Choi and Shin, 2020; Khan et al., 2022; Tang et al., 2024; Torgbo and Sukyai, 2018). Additionally, it possesses desirable characteristics such as high surface area, permeability, porosity, water-holding capacity, biocompatibility, and biodegradability, enabling its application in diverse fields. However, cellulose-based scaffolds, primarily composed of dietary fiber, are limited in providing abundant nutritional value due to their indigestible properties (Wang et al., 2024b). In fact, bacterial cellulose has rarely been utilized as a scaffold biomaterial for cultured meat. A previous study reported that while bacterial nanocellulose can potentially support the formation of mature myotubes by providing surface anchor points, C2C12 myoblasts exhibited limited attachment compared to traditional cell culture plastics over a 3-day incubation period (Rybchyn et al., 2021). This finding suggests potential limitations for its application in cultured meat production. However, within the field of tissue engineering, bacterial nanocellulose has demonstrated significant success in culturing human skeletal muscle myoblasts (Mastrodimos et al., 2024). These cultures exhibit a physiologically similar morphology to myofibers and display superior mechanical properties compared to commercially available matrices. These findings suggest that a deeper understanding of bacterial cellulose could open its potential for wider applications in cultured meat.
Gellan, an anionic polymeric polysaccharide synthesized by Sphingomonas elodea, forms a hydrogel network through cation-mediated helical bonding (Alharbi et al., 2024). During gelation, monovalent cations promote aggregation by suppressing electrostatic repulsions, while divalent cations form direct bridges between pairs of carboxyl groups (Ferris et al., 2013; Moxon and Smith, 2016). Divalent cations, which form stronger gels with gellan than monovalent cations, are primarily used in scaffold fabrication. Conversely, gellan has limited water resistance which makes it suitable for applications as a thickener and gelling agent (Ferris et al., 2013; İncili et al., 2025). Due to its bioinertness and limited cell attachment, gellan is often combined with other biomaterials for scaffold (Koivisto et al., 2019). For example, a previous study successfully addressed the limitations of gellan gum by creating a composite scaffold (Chen et al., 2023b). This scaffold incorporated 1% gellan gum and 0.5% gelatin in a 2:3 ratio and was crosslinked using 0.18 M calcium ions. The results demonstrated significantly improved biocompatibility, including enhanced cell attachment, proliferation, and differentiation of chicken skeletal muscle satellite cells. Additionally, 2% gellan gum was blended with 0.5% and 1% soy or pea protein isolates and these gellan-protein hydrogels exhibited excellent biocompatibility and homogeneous cell encapsulation. Consequently, combining gellan with other biomaterials is crucial to enhance its cell-attachment properties and enable its successful application in scaffold fabrication.
Conclusion
The properties of scaffolds, which play a crucial role in determining the structural, functional, and sensory qualities of cultured meat, are influenced by the type of biomaterials. Biomaterials for scaffolds should possess suitable properties such as structural stability, edibility, and biocompatibility to mimic the muscle tissues of meat. This review highlights the strengths and limitations of individual biomaterials depending on biological sources for fabricating ideal scaffolds in cultured meat production (Fig. 1). However, combining one biomaterial with other biomaterials is essential to address the limitations of individual biomaterials. Therefore, further investigation should focus on the fabrication of scaffolds that support cell culture and mimic muscle tissue through the optimization of biomaterial combinations. Furthermore, scaffold fabrication techniques such as freeze-drying, 3D bioprinting, electrospinning, and electrospray should be selected and optimized according to the type of biomaterials. In conclusion, this review comprehensively examines the properties of biomaterials essential for developing ideal scaffolds for cultured meat production. The successful production of cultured meat necessitates the integration of suitable biomaterials with advanced scaffold fabrication techniques.
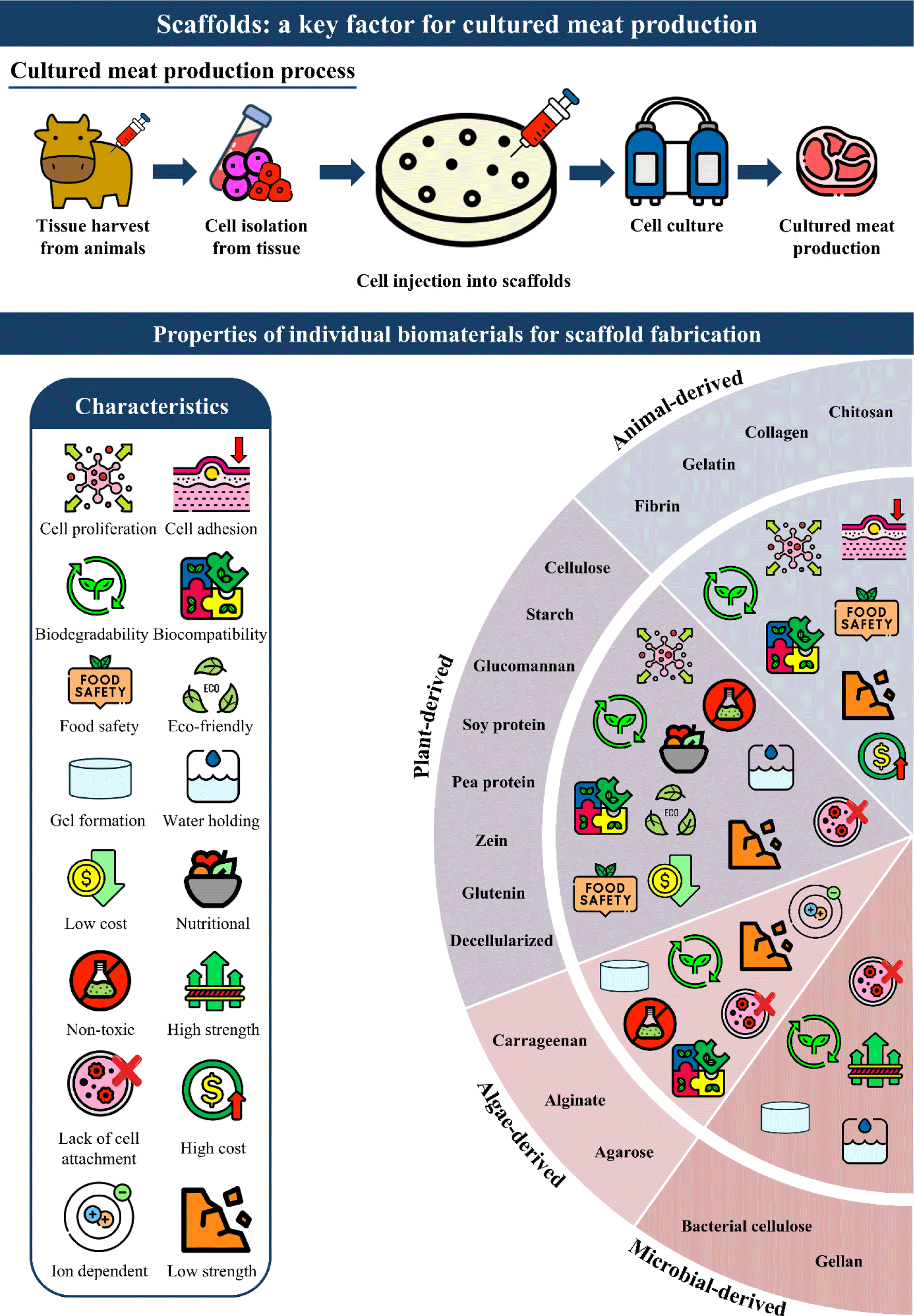