Introduction
Lactic acid bacteria (LAB), regarded as useful probiotics, play a crucial role in fortifying the intestinal barrier against food-borne pathogenic bacteria (Kao et al., 2020; Levit et al., 2019) and modulating intestinal microbiota and immune systems (Levit et al., 2019). LAB have been consumed in various types of foods, including dairy products (Oshiro et al., 2021; Parvarei et al., 2021), fermented fruits and vegetables (Lorn et al., 2021; Oshiro et al., 2021), sourdough (Oshiro et al., 2021), and meat products (Parlindungan et al., 2021). Currently, LAB are used as pharmaceutical agents and not limited to probiotics (Barros et al., 2020). Postbiotics, which are inactivated probiotics (Barros et al., 2020; Parvarei et al., 2021) and their metabolites, have been investigated in a broad spectrum of food and pharmaceutical industries (Barros et al., 2020).
Innate immune system operates as the first-line defense in the host (Lee et al., 2020). Myeloid cells (macrophages, monocytes, and neutrophils) are critical components of innate immunity (Mantovani and Netea, 2020). Myeloid cells recognize pathogen-associated molecular patterns (PAMPs) from infectious microbes and danger-associated molecular patterns from injured tissues caused by Toll-like receptors (TLRs), retinoic acid-inducible gene I-like receptors, nucleotide-binding oligomerization domain-like receptor family proteins, and absent in melanoma 2, a family of pattern-recognition receptors (PRRs; Lee et al., 2020). Innate immune system activates macrophages and immediately counteracts to pathogens to provide host defenses against various types of invaders (Geng et al., 2018; Jeong et al., 2019; Lee et al., 2020; Leopold Wager and Wormley, 2014; Liu et al., 2019; Mantovani and Netea, 2020; Netea et al., 2020; Um et al., 2020). Unlike these rapid reactions, the trained immune system involves reprogramming of innate immune cells awakened by exogenous or endogenous stimulations (Netea et al., 2020). For example, monocytes that were first treated with β-glucan lost stimulus within 24 h, and the second challenge by lipopolysaccharides (LPS) showed a burst of tumor necrosis factor-α (TNF-α) and interleukin-6 (IL-6; Bauer et al., 2018). These well-trained cells undergo epigenetic modifications and have long-term memory effects (Bauer et al., 2018; Netea et al., 2020).
Lactiplantibacillus plantarum is a facultative heterofermentative Lactobacillus species (Liu et al., 2018). The European Food Safety Authority (EFSA) has acknowledged L. plantarum as a Qualified Presumption of Safety (QPS) and has continuously updated its strains since 2007 (Andreoletti et al., 2008; Liu et al., 2018). L. plantarum has been used not only for starter culture in the food industry (Le and Yang, 2018; Liu et al., 2018) but also in pharmacological research owing to its bio-functionalities (Le and Yang, 2018).
The aim of this study is investigation of immunostimulatory effects of heat-treated L. plantarum LM1004 (HT-LM1004). Other studies have been focused on the immunostimulatory effects of probiotics and their metabolites, such as exopolysaccharides, whereas heat-treated probiotics are not of interest. It had been known that heat treatment disrupts the bacterial cell wall and induces release of nucleic acid, peptidoglycan, and teichoic acids, resulting in modulating immune responses by these strain specific bacterial components (Piqué et al., 2019). Our previous study, micronized and heat-treated L. plantarum (MHT-LM1004) and HT-LM1004 showed increase of natural killer (NK) cell activity and relative cytokine production in immune-suppressed mice (Jung et al., 2019). However, molecular level mechanisms of immunostimulatory effect of HT-LM1004 were not fully understood. In addition, MHT-LM1004 is defined as similar material due to manufacturing methods and is not suitable for industrial production. Therefore, the immunostimulatory effects of HT-LM1004 via the mitogen-activated protein kinase (MAPK)/Activator protein 1 family (AP-1)/Nuclear factor kappa B (NF-κB) pathway were investigated in this study.
Materials and Methods
LPS from E. coli O111:B4 (LPS) and ammonium pyrrolidine dithiocarbamate (APDC) were purchased from Sigma-Aldrich (St. Louis, MO, USA). Thiazolyl Blue tetrazolium bromide (MTT) was obtained from Alfa Aesar (Haverhill, MA, USA). Antibodies against cyclooxygenase-2 (COX-2), phospho-p38 MAPK, p38 MAPK, phospho-extracellular signal-regulated kinase 1/2 (ERK1/2), ERK1/2, phospho-c-Jun N-terminal kinase (JNK), JNK, c-Fos, c-Jun, phospho-IκBα, IκBα, phospho-p65 NF-κB, p65 NF-κB, phospho-AMP-activated protein kinase (AMPK), AMPK, phospho-acetyl-CoA carboxylase (ACC), ACC, and glyceraldehyde-3-phosphate dehydrogenase were obtained from Cell Signaling Technology (Danvers, MA, USA). The anti-inducible nitric oxide synthase (iNOS) antibody was obtained from GeneTex (Irvine, CA, USA).
L. plantarum LM1004 was isolated from kimchi, Korean traditional fermented food. In brief, 25 g of kimchi was homogenized in 225 mL of phosphate buffered saline (PBS) using a stomacher. After homogenization, sample was diluted in peptone water (0.1%, w/v) and spread on de Man-Rogosa-Sharpe (MRS) agar (for Lactobacillus; BD, Franklin Lakes, NJ, USA), M17 agar (for lactic Streptococcus and Lactococcus; MBcell, Seoul, Korea), and Bifidobacterium selective agar (Bifidobacterium spp.; MBcell). The spread agar plates were incubated at 37°C for 48 h. After 48 h, colonies isolated from MRS agar were spread on Bromocresol purple (BCP) agar and yellow colonies on BCP agar further purified in newly prepared MRS agar until single colony. Single and pure colony was enriched in MRS broth for gram-staining and catalase reaction. The isolate was identified gram-positive and catalase-negative strain with rod-type shape. Isolated strain was named LM1004 and identified by 16S rRNA sequencing as L. plantarum. L. plantarum LM1004 was stored in MRS containing with 20% glycerol at –80°C until use (Ngamsomchat et al., 2022).
For analysis of complete genome sequencing, genomic DNA (gDNA) of L. plantarum LM1004 was extracted by TaKaRa MiniBEST Bacteria Genomic DNA Extraction Kit (Takara Bio, Kusatsu, Japan). The DNA sequencing library was constructed using single molecular real-time sequencing technology (Pacific Biosciences, Menlo Park, CA, USA). De novo assembly was performed using Celera Assembler in hierarchical genome assembly process (Macrogen, Seoul, Korea).
HT-LM1004 was obtained from the Department of Production, Lactomason (Jinju, Korea). The cell numbers and morphology were constantly managed by the Quality Management Team (Lactomason). Lyophilized heat-treated cells were assigned the product number 11NTF8 and stored at –20°C until use.
Extraction of cellular fatty acid from HT-LM1004 was performed by Bligh and Dyer method with modification (Cheng et al., 2022). Briefly, 200 μL of chloroform/methanol solution (2:1, v/v) and 300 μL of 0.6 M hydrochloric acid solution (in methanol) were added in 20 mg of HT-LM1004. The mixture was shaken for 2 min vigorously and heated at 85°C during 60 min. The extracted lipids were cooled at 25°C for 20 min. Fatty acid methyl esters (FAME) were more extracted by n-hexane for 60 to 120 min. The FAME extracted layer (n-hexane layer) was transferred into clear vial and stored at –20°C until analysis.
Cellular fatty acid analysis was performed by gas chromatography–mass spectrometry detection (GC/MSD). The GC/MSD system was composed of Agilent 8890 gas chromatography system coupled with a 5977B mass selective detector and 7693A automated liquid sampler (Agilent, Santa Clara, CA, USA). An Agilent J&W DB-FastFAME capillary column packed with cyanopropyl (30 m×0.25 mm, 0.25 μm) was employed. Injection port temperature was 250°C in constant flow and 1 μL of sample was injected using the spilt mode of 20:1. Ultrapure helium gas was used as carrier gas with a flow rate of 1 mL/min. The initial oven temperature was retained at 60°C for 1 min, raised from 60°C to 165°C at a rate of 60°C/min, held 1 min at 165°C, raised form 165°C to 230°C at a rate of 5°C/min, and kept for 3 min. The temperature of ion source and transfer line was 230°C, and 250°C, respectively. The mass spectra were obtained on an electron ionization at 70 eV and recorded m/z 40–550 of mass range. Methyl undecanoate was used as internal standard (Liu et al., 2022).
The murine macrophage cells, RAW 264.7 cell lines, were obtained from the Korean Cell Line Bank (KCLB, Seoul, Korea). The cells were maintained in Dulbecco’s modified Eagle’s medium (DMEM) supplemented with 10% fetal bovine serum and 1% penicillin-streptomycin solution at 37°C in a humidified atmosphere containing 5% CO2. When the cells were grown to 80% confluence, they were gently harvested using a scraper. Harvested cells were seeded in various well plates and incubated for 24 h. After incubation, cells were treated with LPS (10 ng/mL) or HT-LM1004 to measure immunostimulatory and phagocytic effects (Liu et al., 2019). Cells were pre-treated with APDC, an NF-κB inhibitor, for 1 h before treatment with LPS or HT-LM1004.
Macrophage cells were seeded in a 96-well microplate (1×105 cells/well) and incubated for 24 h. After incubation, each well was treated with LPS or HT-LM1004 (1×107, 2.5×107, 5×107, and 1×108 cells/mL) and further incubated for 24 h. The incubated cells were washed with PBS twice times and fresh DMEM including 0.5 mg/mL of MTT was used to replace any lost medium (Um et al., 2020). The absorbance of MTT formazan which viable cell converted was evaluated at 570 nm using a microplate reader (SpectraMax iD3, Molecular Devices, San Jose, CA, USA). Cell viability was calculated as follows:
where Asample is the absorbance of LPS or HT-LM1004 treated cells and Acontrol is the absorbance of non-treated cells (negative control).
The NO content was measured using Griess reagent (Geng et al., 2018). Briefly, RAW 264.7 macrophage cells (1×105 cells/well) were treated with LPS (positive control) or HT-LM1004 for 24 h. After 24 h, cell-free supernatants were collected, and added Griess reagent (Promega, Madison, WI, USA) for measuring NO contents according to the manufacturer’s guidelines.
The release of immunostimulatory cytokines (TNF-α and IL-6) was measured using an enzyme-linked immunosorbent assay (Bo et al., 2019; Liu et al., 2019). Cell culture and sample treatments were prepared as described in cell culture and treatment. Cell-free supernatants were collected by centrifugation at 1,000×g for 20 min at 4°C. All immunostimulatory cytokines were analyzed according to the manufacturer’s guidelines (Invitrogen, Waltham, MA, USA).
The phagocytic effect of HT-LM1004 treated RAW 264.7 macrophage cells was evaluated using enzyme-labeled E. coli particles (CytoSelectTM 96-Well Phagocytosis Assay, Cell Biolabs, San Diego, CA, USA; Jeong et al., 2019). Relative phagocytic effects were measured by enzyme-substrate reactions, according to the manufacturer’s guidelines.
The HT-LM1004 treated RAW 264.7 macrophage cells were lysed by RIPA lysis buffer (containing 50 mM Tris-HCl, 150 mM NaCl, 1% Triton-X, 1% sodium deoxycholate, 0.1% SDS and 2 mM EDTA) with protease and phosphatase inhibitor cocktail (Thermo Fisher Scientific, Waltham, MA, USA). The lysed cells were centrifuged at 13,000×g for 20 min at 4°C. The supernatants were collected, and protein content was measured using the PierceTM BCA Protein Assay Kit (Thermo Fisher Scientific). The extracted proteins were stored at 4°C until further use.
Proteins were separated by capillary western blot analysis (Khan et al., 2021) or sodium dodecyl sulfate-polyacrylamide gel electrophoresis (SDS-PAGE). For capillary western blotting, proteins were diluted to 0.8 mg/mL and separated by a 12–230 kDa capillary cartridge (ProteinSimple, San Jose, CA, USA) according to the manufacturer’s guidelines. Protein separation and immunodetection were conducted using JESS, an automated western blotting system (ProteinSimple).
For SDS-PAGE, proteins (30 μg) were separated to 8% or 10% of SDS-PAGE gel and transferred onto polyvinylidene fluoride membranes. Membranes were blocked with 3.75% skim milk for 1 h, followed by incubation with primary antibodies at 4°C for 24 to 48 h. After incubation, the membranes were washed with Tris-buffered saline containing Tween 20 and incubated with secondary antibodies at 25°C for 2 h. The blots were visualized using ECL detection reagent (Advansta, San Jose, CA, USA). The intensity of the bands was analyzed using ImageJ software.
Results
The whole genome characteristics of L. plantarum LM1004 are shown in Fig. 1A. The size of entire gene sequence of L. plantarum LM 1004 was 3,198,690 bp, single and circular chromosome with 44.59% of GC content. A total of 3001 protein-coding sequences were identified in L. plantarum LM1004. The chromosome include 16 rRNA and 68 tRNA. The complete genome sequence of L. plantarum LM1004 has been deposited in the National Center for Biotechnology Information GenBank database under the accession number CP025988.
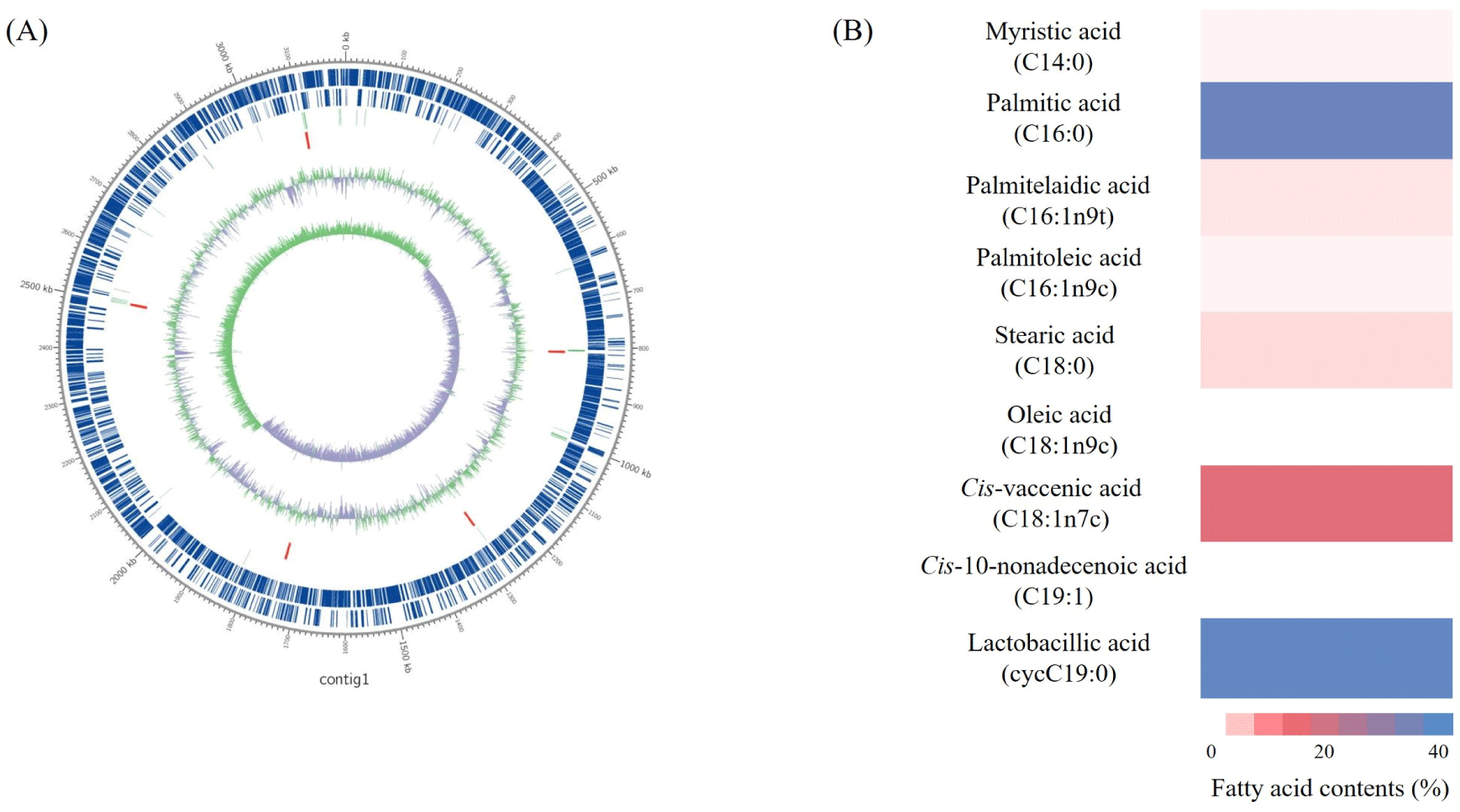
Cellular fatty acid composition of HT-LM1004 are shown in Figs. 1B and C. Total 7 kinds of fatty acid were observed in HT-LM1004. Lactobacillic acid (cycC19:0) and palmitic acid (C16:0) were investigated most abundant cellular fatty acid in HT-LM1004. The proportion of saturated fatty acid (SFA), unsaturated fatty acid (USFA) and cyclic fatty acid (CFA) in HT-LM1004 were measured 41.42%, 20.03%, and 38.55%, respectively.
Prior to measuring the immunostimulatory effects of HT-LM1004, cell viability was investigated using MTT formazan. Cytotoxicity was not shown in HT-LM1004 or LPS treated (positive control) macrophage cells (Fig. 2A). The NO content and iNOS expression are shown in Figs. 2B and C. The HT-LM1004 (1×107, 2.5×107, 5×107, and 1×108 cells/mL) treated RAW 264.7 macrophage cells released 3.05, 7.55, 12.55, and 16.32 μM of NO, respectively (p<0.01). The relative expression of iNOS increased 6.59-, 14.24-, 17.14-, and 19.86-fold compared to non-treated RAW 264.7 macrophages (negative control; p<0.01).
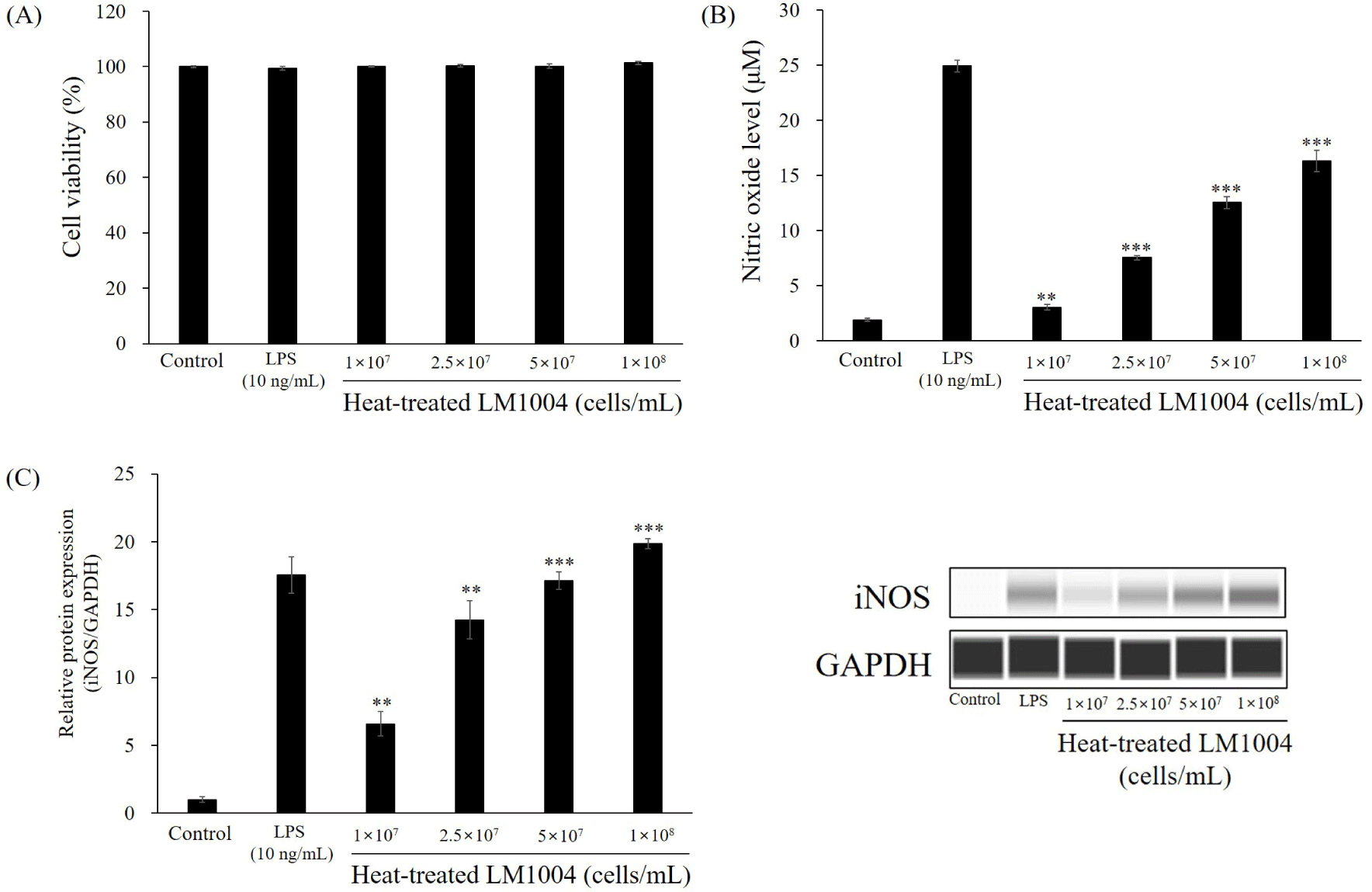
The release of immunostimulatory cytokines (TNF-α and IL-6) and relative protein expression of COX-2 are shown in Fig. 3. HT-LM1004 increased TNF-α secretion from 205.52 (negative control) to 1,530.11, 1,925.27, 3,445.44, and 3,906.01 pg/mL (p<0.01). In addition, HT-LM1004 treated RAW 264.7 macrophages released 254.36, 302.66, 394.29, and 651.93 pg/mL of the immunostimulatory cytokine IL-6 (p<0.01). The relative protein ex-pression of COX-2 was up-regulated in HT-LM1004 treated RAW 264.7 macrophage cells. COX-2 expression increased to 25.25-fold at 1×108 cells/mL of HT-LM1004 treated RAW 264.7 macrophage cells (p<0.001; Fig. 3C).
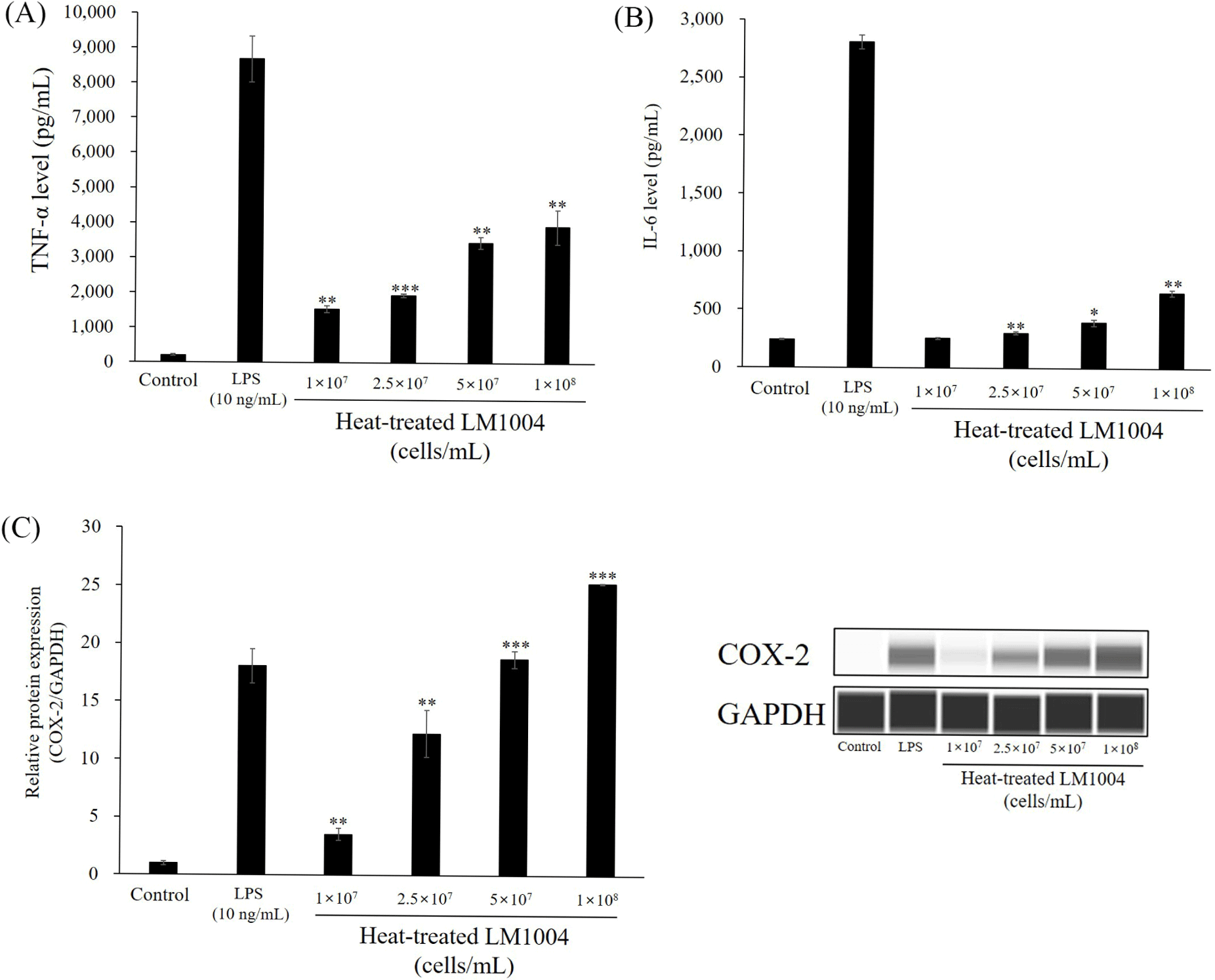
Figs. 4 and 5 present changes in MAPK and transcription factor in HT-LM1004 treated RAW 264.7 macrophage cells. HT-LM1004 treated RAW 264.7 macrophage cells were used to investigate the phosphorylation of MAPK sub-families (p38, ERK1/2, and JNK). Briefly, phosphorylation of p38 MAPK, ERK1/2, and JNK increased to 4.96-, 5.52-, and 2.98-fold at 1×108 cells/mL of HT-LM1004 treated macrophage cells (p<0.05). Moreover, phosphorylation of IκBα and activation of NF-κB p65 translocation were observed in HT-LM1004 treated cells (Fig. 5; p<0.01). Other transcription factors (c-Fos and c-Jun) also increased protein expression (p<0.05).
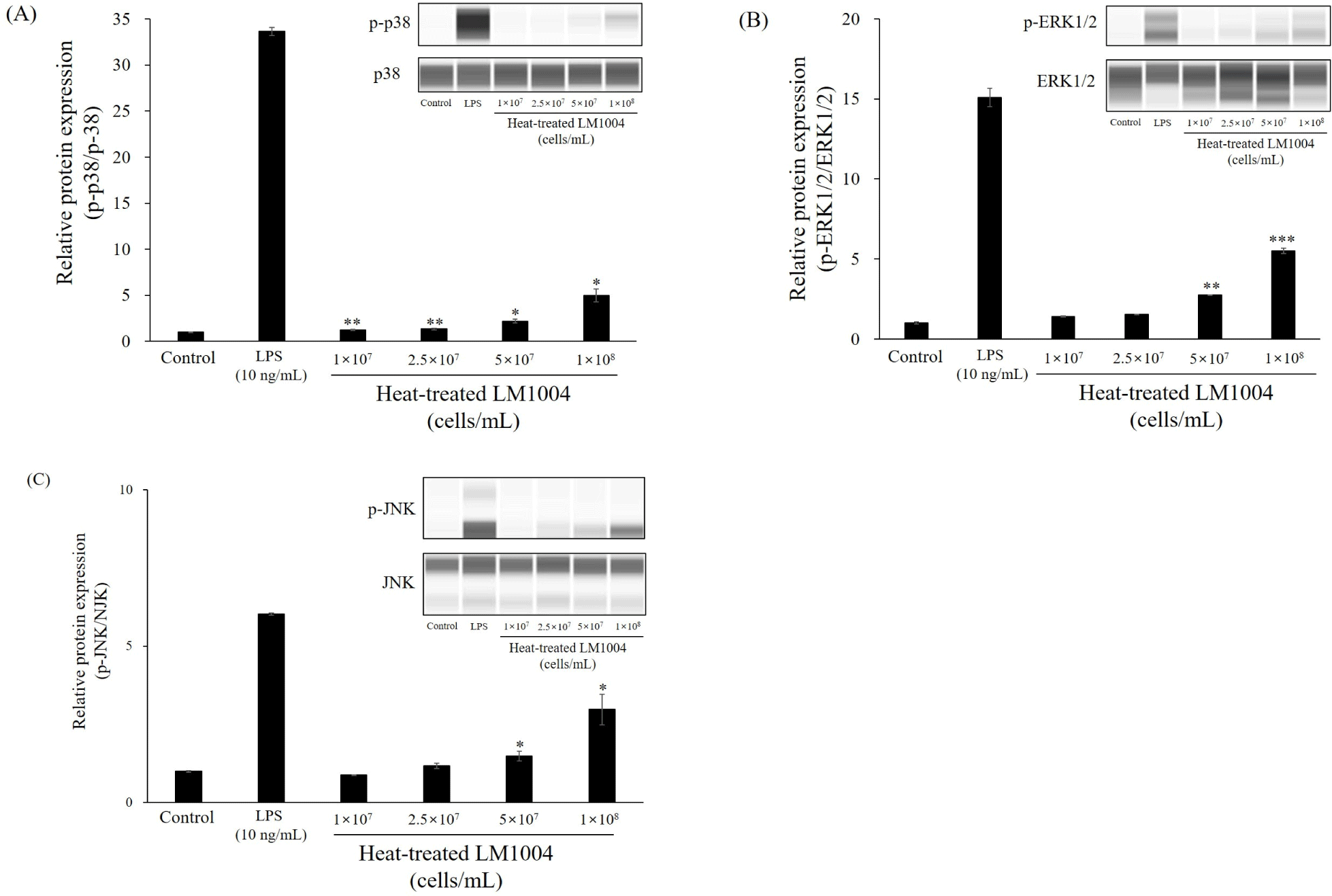
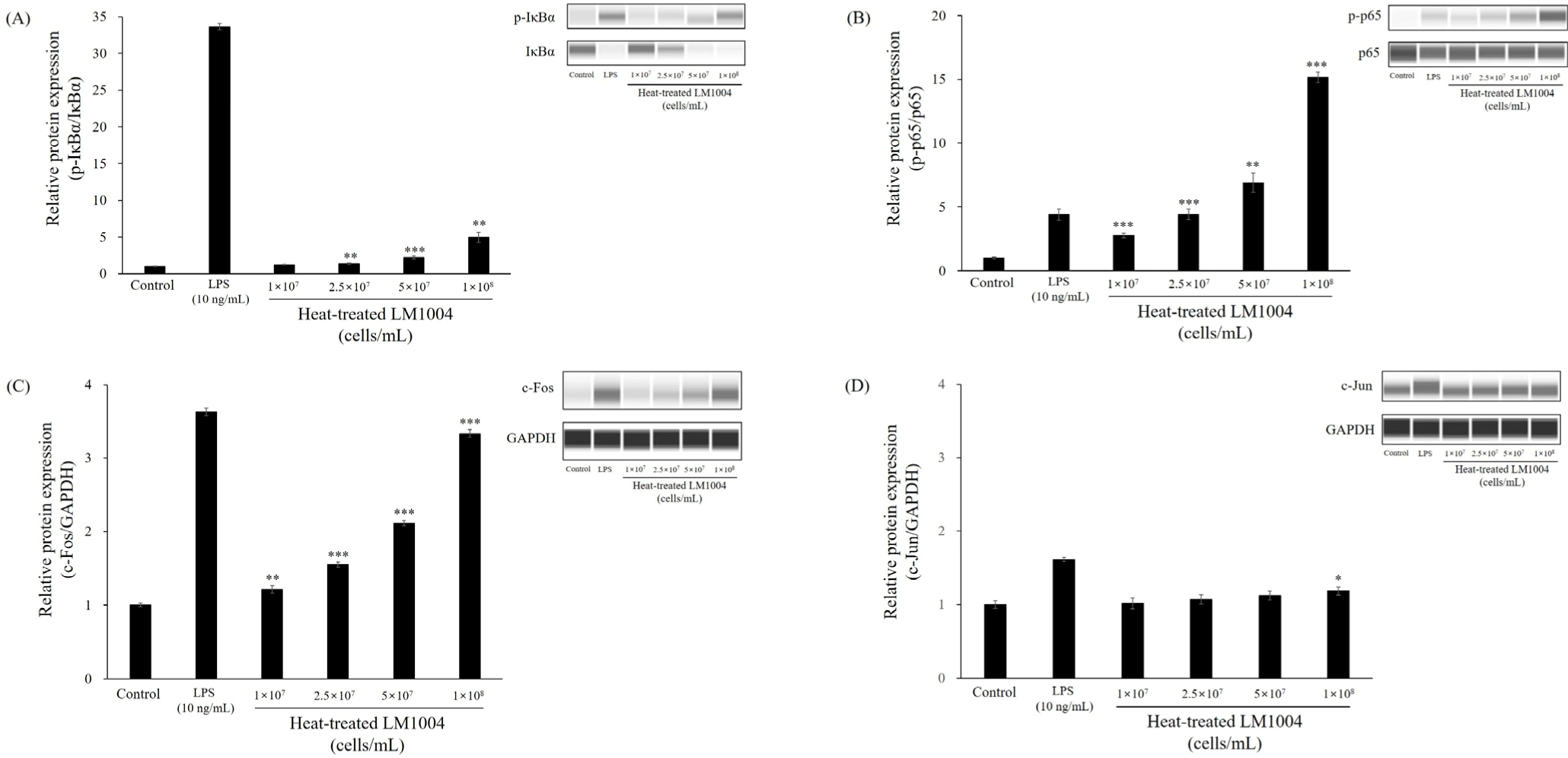
APDC, a pharmacological NF-κB inhibitor, prevents iNOS expression and NO pro-duction (Dong et al., 2015). In the current study, the immunostimulatory effects of HT-LM1004 were investigated by the upregulation of iNOS and the release of NO in APDC-treated RAW 264.7 macrophage cells. The APDC-treated cells inhibited the release of NO (3.81 μM) though HT-LM1004 treated cells produced 4.46 and 7.31 μM of NO at 5×107 and 1×108 cells/mL, respectively (p<0.01). The APDC and HT-LM1004 co-treated cells also showed an over-expressed iNOS level comparing to non-treated RAW 264.7 macrophage cells (p<0.001; Fig. 6).
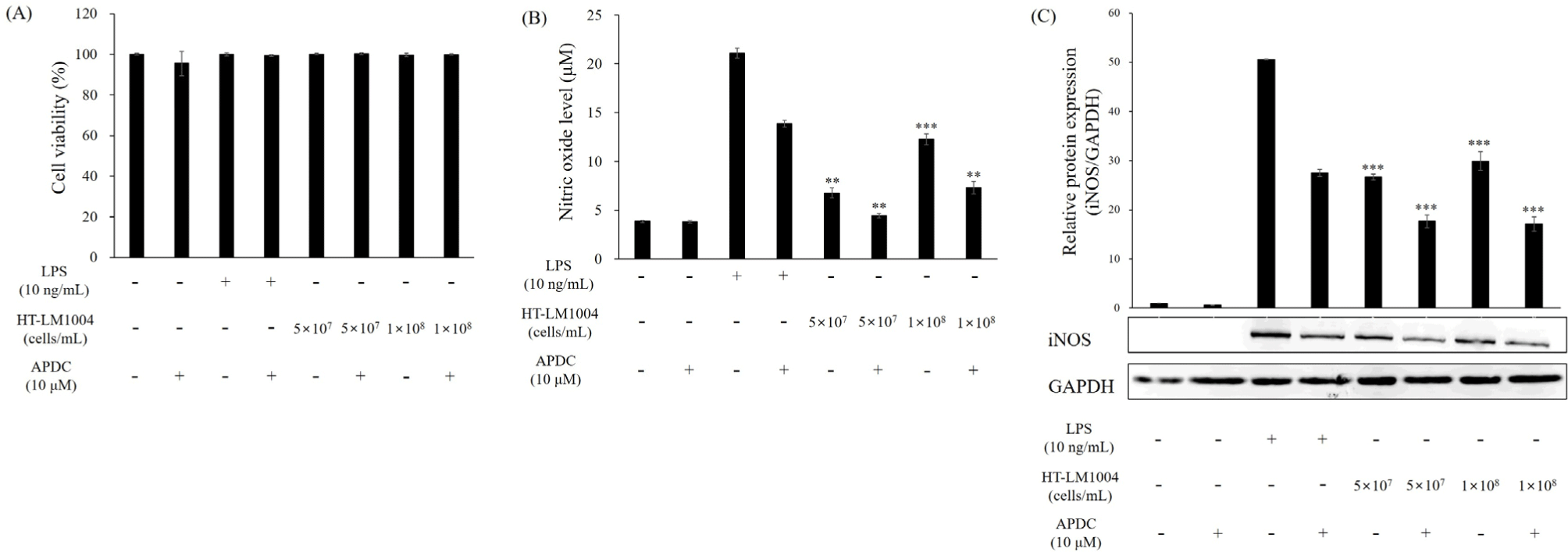
The phagocytic effect of HT-LM1004 treated cells is shown in Fig. 7. The 1×107 cells/mL of HT-LM1004 treatment increased phagocytosis of macrophage cells (123.18%), but no significant differences were detected. Phosphorylation of AMPK and ACC did not significantly change in the HT-LM1004 treated macrophages.
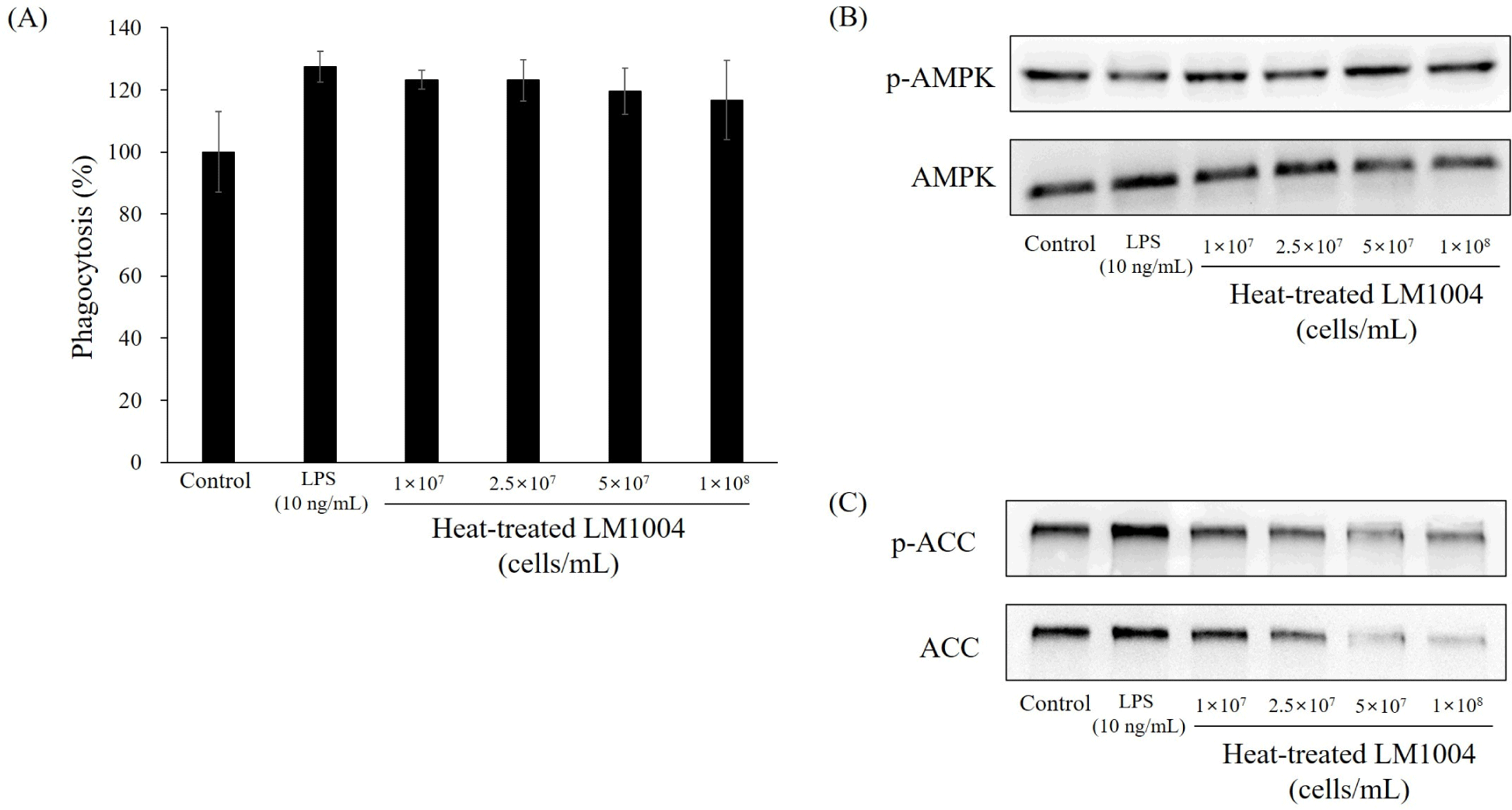
Discussion
The interactions between LAB and the host immune system have not been clearly reported, but many researchers have suggested that PRRs recognize LAB cell wall-derived molecules as PAMPs (Ren et al., 2020). Lipoteichoic acid (LTA), the most representative cell wall-derived PAMP in gram-positive bacteria, is an important ligand for innate immune responses (Friedrich et al., 2022; Jung et al., 2022; Kang et al., 2011; Ren et al., 2020). LTA is an amphiphilic molecule with both a hydrophilic polysaccharide moiety and a hydrophobic glycolipid region (Kang et al., 2011). In general, LTA interacts with TLR2, which is associated with myeloid differentiation primary response 88 (MyD88), interleukin-1 receptor-associated kinases, and TNF receptor-associated factor 6 (Jung et al., 2022). These TLR2-MyD88 dependent signaling pathways upregulate release of immunostimulatory cytokines and chemokines (Kang et al., 2011). The immunogenicity of LTA depends on its structural diversity in accordance with the genus and species levels (Friedrich et al., 2022). Ryu et al. (2009) reported that LTA isolated from three different gram-positive bacteria (Staphylococcus aureus, Bacillus subtilis, L. plantarum) showed relative differences in NF-κB translocation and TNF-α secretion. Moreover, Jung et al. (2022) reported differential immunostimulatory effects of LTA isolated from four different strains of L. plantarum and analyzed differences in glycolipid composition. Considering the immunogenicity of LTA, heat-treated LAB also showed immunomodulatory effects of LTA. In the current study, HT-LM1004 induced the release of immunostimulatory cytokines (TNF-α and IL-6; Fig. 3) and translocation of NF-κB (Fig. 5). These results were also observed in other heat-treated L. plantarum species (Choi et al., 2018; Jeong et al., 2019; Moon et al., 2019). In addition, Kim et al. (2018) reported that heat-treated LAB contributed immunomodulatory food additives and prolonged the shelf life.
NO is synthesized in various cells for neurotransmission, vascular function, host defense, and immune regulation (Xue et al., 2018). NO synthases are classified into three subtypes: Neuronal NO synthase, endothelial NO synthase, and iNOS. In particular, iNOS is mainly expressed in immune-stimulated cells by cytokines and inflammatory molecules (PAMPs) such as LPS and LTA (Kang et al., 2011; Xue et al., 2018). NO plays a critical role in the regulation of M1 macrophage polarization. M1 macrophages are able to respond to pro-inflammatory responses and produce cytokines such as TNF-α, IL-6, and IL-12 for host defense (Yunna et al., 2020). Additionally, NO generated by iNOS expression in M1 macrophages directly defends against pathogens (Xue et al., 2018). HT-LM1004 induced release of NO levels and expressed iNOS in RAW 264.7 macrophage cells (Fig. 2). HT-LM1004 also affected the release of immunostimulatory cytokines (Fig. 3).
NF-κB signaling is crucial in physiological processes. NF-κB transcription factors are involved in cellular transformation and proliferation, apoptosis, angiogenesis, metastasis, and activation of the immune system (Aggarwal, 2004). In the immune system, the NF-κB transcription factor is involved in inflammatory responses to microbes and viruses by innate immune cells and the development of adaptive immune cells in secondary lymphoid organs (Dorrington and Fraser, 2019). IκBα degradation and phosphorylation activates the NF-κB transcription factor (p65) from the cytoplasm to the nucleus (Geng et al., 2018). The translocation of NF-κB mediates the transcription of immunostimulatory molecules and cytokines, including iNOS, COX-2, TNF-α, IL-2, IL-6, and IL-12 (Geng et al., 2018; Moon et al., 2019; Yang et al., 2019). TNF-α, IL-2, and IL-12 contribute to the activation of NK cells, which play a crucial role in the host defense system against pathogens and transformed cells (Lauwerys et al., 2000; Moon et al., 2019). These cytokines promote cytotoxicity of NK cells and immunomodulatory effects of NK cells in the innate and adaptive immune systems (Lauwerys et al., 2000). In addition, Sharma and Das (2018) reported that IL-2 mediates the proliferation of NK cells.
The MAPK cascade promotes the transcription of transcriptional factors, such as NF-κB and AP-1 (Geng et al., 2018; Liu et al., 2019; Yang et al., 2019). AP-1 consists of four subfamilies: Jun, Fos, ATF-activating transcription factor protein families and musculoaponeurotic fibrosarcoma. AP-1 in immune system has been reported to play a role in Th differentiation, T-cell activation, and T-cell anergy (Atsaves et al., 2019). Activation of the MAPK pathway via a cascade of phosphorylation events on serine/threonine residues coordinates downstream of AP-1 and NF-κB (Atsaves et al., 2019; Geng et al., 2018). Thus, MAPK activation by HT-LM1004 plays a central role in the innate immune system.
Phagocytosis is occurred in three classes of phagocytic cells in immune system such as monocytes/macrophages, neutrophilic granulocytes and dendritic cells (Schumann, 2016). Macrophages act as scavenger of pathogens, dead cells, and debris. When macrophages engulf pathogens, phagosomes are fused with lysosomes which result in phagolysosomes and toxic peroxides for digesting the pathogens (Jeong et al., 2014; Schumann, 2016). Fatty acids can influence modulating of immune response of macrophages including phagocytosis and cytokine productions (Schumann, 2016). Calder et al. (1990) reported SFA such palmitic acid result in decrease of phagocytosis of macrophages. On the other hand, USFA increased phagocytosis of macrophages except oleic acid (C18:1). However, palmitic acid activate TLR-MyD88 dependent NF-κB activation and production of immunostimulatory cytokines (Korbecki and Bajdak-Rusinek, 2019). In current study, the contents of SFA were measured 2-times higher than USFA in cellular fatty acid of HT-LM1004. Palmitic acid is a most abundant fatty acid except for lactobacillic acid which is CFA (Figs. 1B and C). The 1×107 cell/mL of HT-LM1004 treated cells showed highest phagocytosis effect (123.18%) while 1×108 cell/mL treated macrophage cells decreased to 116.69% (Fig. 7). However, palmitic acid derived from cellular membrane of HT-LM1004 induced immunostimulatory effects by activation of NF-κB (Figs. 5 and 6).
Conclusion
In the present study, the immunostimulatory potency of HT-LM1004 was investigated at various stages of innate immunity. HT-LM1004 stimulated the MAPK pathway and regulated transcription factors, such as AP-1 (c-Fos and c-Jun) and NF-κB p65. These transcription factors induce secretion of NO, TNF-α, and IL-6 to enhance the immune system. Heat-treated LAB lost their probiotic properties, but HT-LM1004 showed immunostimulatory effects as a postbiotic. These results suggest HT-LM1004 as an immunostimulatory agent, food additive, and therapeutic agent.